1. INTRODUCTION
Astronomical studies have shown that the materials originated from the Standard Model (SM) accounts for only 4% of the components of the universe (Cho 2016; Yeo & Cho 2018). Though the SM of particle physics agrees well with experimental results, the SM does not provide an information for dark matter, which is the dominant matter component (26%) of the universe (Cho 2016; Yeo & Cho 2018). Because dark matter only interacts gravitationally with SM particles, it is difficult to detect it directly. Dark matter is known to play an important role in the evolution of the universe in constructing cosmic structures including stars, galaxies, and galaxy clusters. Furthermore, the presence of dark matter suggests the existence of the “beyond the SM” (BSM). Therefore, identifying dark matter is an important issue in modern particle physics and cosmology.
Dark matter has not been directly detected to date because its interaction with ordinary matter is very weak. Nevertheless, three types of experimental methods have been actively conducted to search for dark matter (Cho 2017; Yeo & Cho 2018). One is a direct search method used to study the signals generated by dark matter colliding with the SM particles. Another experiment is an indirect search method that observes excess gamma rays produced by the pair annihilation of dark matter in the center of the galaxy, where abundant dark matter is distributed. Finally, there is an accelerator search method that uses accelerators to study the signals of dark matter or dark photons produced from the SM particles (Ilten et al. 2016; Aaij et al. 2018; He et al. 2018; Hearty 2022; San et al. 2022).
In this paper, we studied dark photons. Dark photons are hypothetical particles that have similar role to photons in electromagnetic interaction but have been proposed as force carriers with a potential link to dark matter (Park et al. 2021a). We have done simulation studies with a parametrized response of the detector simulation of Delphes. We presented the expected number of signal events in each experiment with the detector efficiency. We studied double dark photon decay modes using future electron-positron accelerators such as Circular Electron Positron Collider (CEPC)/CEPC, Future Circular Collider (FCC-ee)/Innovative Detector for Electron-positron Accelerator (IDEA), and International Linear Collider (ILC)/International Large Detector (ILD).
There are two types of accelerator-based experiments. One is based on the hadron accelerator, including the Large Hadron Collider (LHC), which exploits a proton-proton collision. The other is the lepton accelerator which uses an electron-positron collision. Fig. 1 compares the Feynman diagram of the Simplified Model for a hadron accelerator and a lepton accelerator. These two accelerators play a complementary role in the exploration of dark matter. However, electron-positron accelerator provides several favorable research environments (Yeo & Cho 2018). It has the advantage of exploring light dark matter, which has a mass range of a few MeV–GeV. In addition, it provides clean signal events and low background events compared with the hadron accelerator. As future electron-positron accelerators, there are CEPC, FCC-ee, and ILC. We study for dark sector using these electron-positron colliders. Table 1 shows parameters of electron-positron accelerators with detectors (Particle Data Group et al. 2020).
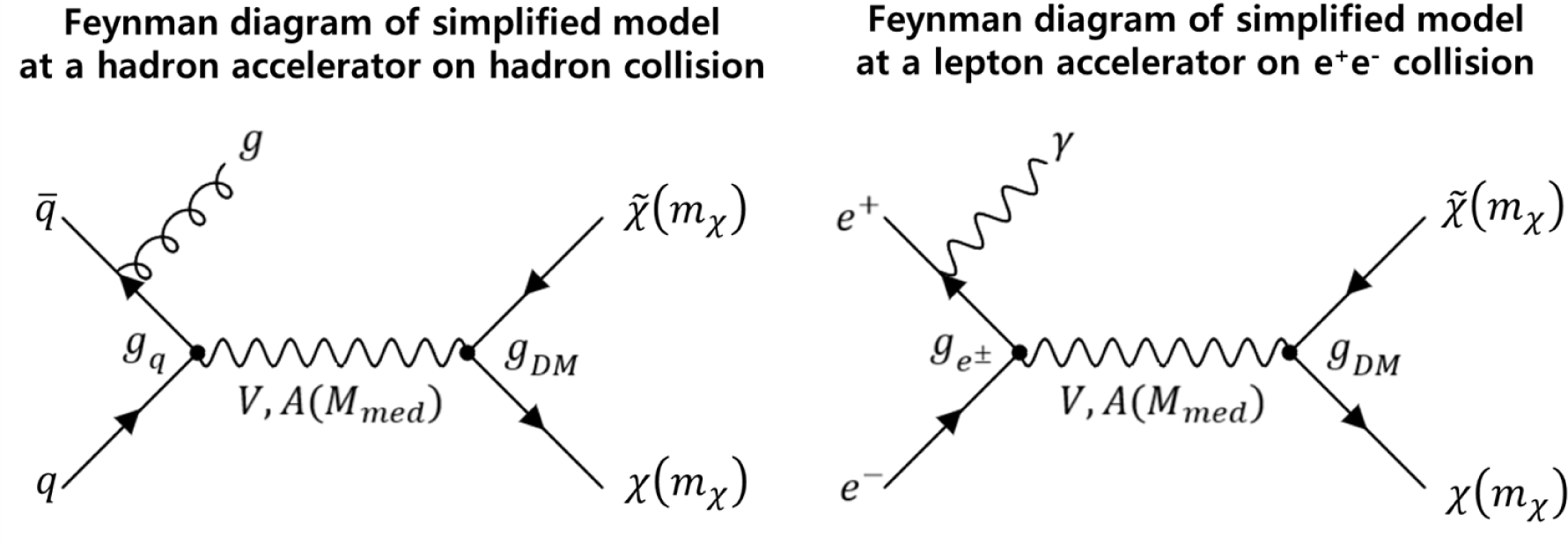
Adapted from Particle Data Group et al. (2020) with permission of the Oxford University Press.
2. THEORY
Because the SM cannot account for dark matter, theoretical physicists assume that there is a dark sector containing dark matter (Shuve & Yavin 2014). We also assume that there are mediator particles between the SM and dark sector. In this paper, we consider a dark photon as a mediator particle. According to theory (Shuve & Yavin 2014), dark photons can only couple to muons. Based on this theory, we studied a double dark photon mode with future electron-positron accelerators. The signal mode is e+e− → A′A′ where each A′ (dark photon with spin 1) decays into dimuon. That is, the final state particles are four muons for the decay mode of e+e− → A′A′. Fig. 2 shows a dominant Feynman diagram of the double dark photon mode. is the coupling between the electron and dark photon. is the coupling between the muon and dark photon. Table 2 summarizes the coupling constants.
Paper | MadGraph5 | Value | Description |
---|---|---|---|
gvl11 | 0.1 | Electron-Y1 vector (A′) coupling | |
gvl22 | 0.1 | Muon-Y1 vector (A′) coupling |
To study dark matter, we used Simplified Model (Morgante 2018). This model is minimally extended from the SM. It includes not only SM particles but also dark matter and mediators, including dark photons. The Simplified Model is located between the ultraviolet and the effective field theory models (Morgante 2018). Originally, this model was used for hadron collider experiments, including the LHC at European Organization for Nuclear Research (CERN) (Abdallah et al. 2015). However, we modified and utilized this model for electron-positron accelerators. Signal events were generated based on the model using MadGraph5 (Alwall et al. 2014).
3. METHODS
In step 1, we generated events using MadGraph5 (Alwall et al. 2014). In event generation, physical parameters of the and dark photon mass had to be scanned, which required a large amount of simulation. Therefore, events were efficiently generated by utilizing the KISTI-5 supercomputer (Park & Cho 2021a, b). In step 2, a detector simulation was performed using Delphes (de Favereau et al. 2014) as the fast simulation. When simulating the detector, Delphes cards (Delphes 2014) were used in each experiment. In step 3, the physical quantities were reconstructed using C++ code and ROOT (Brun & Rademakers 1997). Finally, in step 4, the histogram as a result of reconstruction were fitted with RooFit (Verkerke & Kirkby 2003) to obtain the expected number of signal events.
4. RESULTS
Signal modes include parameters of and dark photon mass, which affect cross-section. Therefore, it is necessary to investigate the cross-section according to and dark photon mass. In this section, we scanned the cross-section for . Table 3 lists the specification of the scan for center-of-mass energy. Fig. 3 shows the center-of-mass energy dependency of the cross-section in the decay mode of e+e− → A′A′ (Park et al. 2022). Red vertical lines indicate individual of accelerators. Cross-section increases as the decreases.
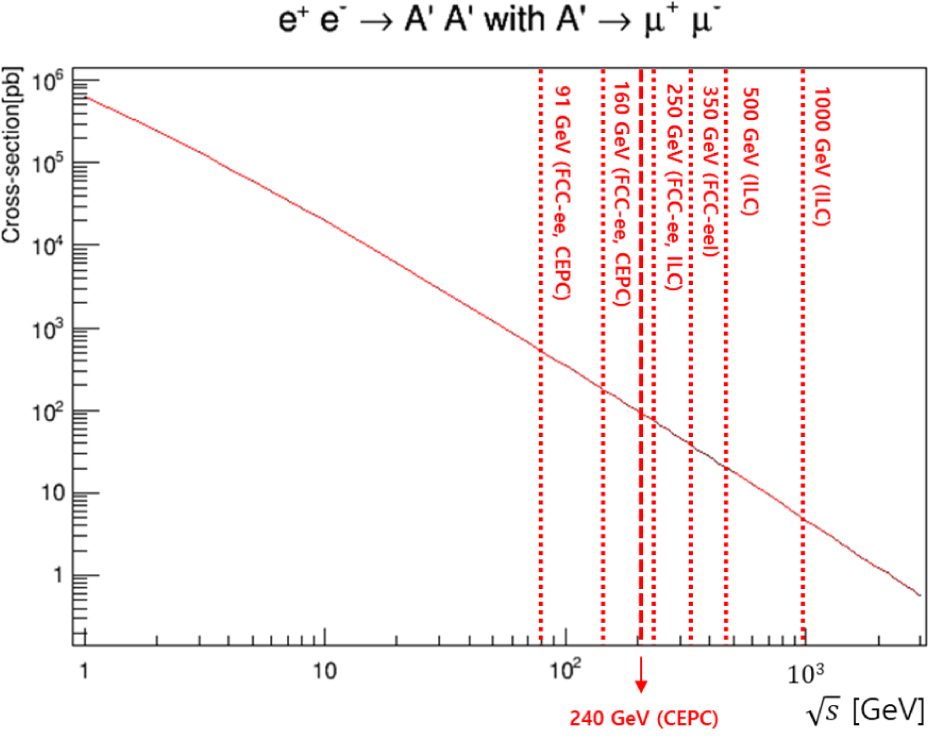
The cross-section was scanned for the dark photon mass from 0.25 to 500 GeV. Table 4 lists the specification of the scan for dark photon mass. Fig. 4 shows the dark photon mass dependency of the cross-section in the decay mode of e+e− → A′A′ at each accelerator. As a result, we found dark photon mass with the largest cross-section at each accelerator energy. And then, we applied these dark photon masses to generate signal events for each experiment. Table 5 shows the dark photon masses of e+e– → A′A′ decay mode with the maximum cross-section at each .
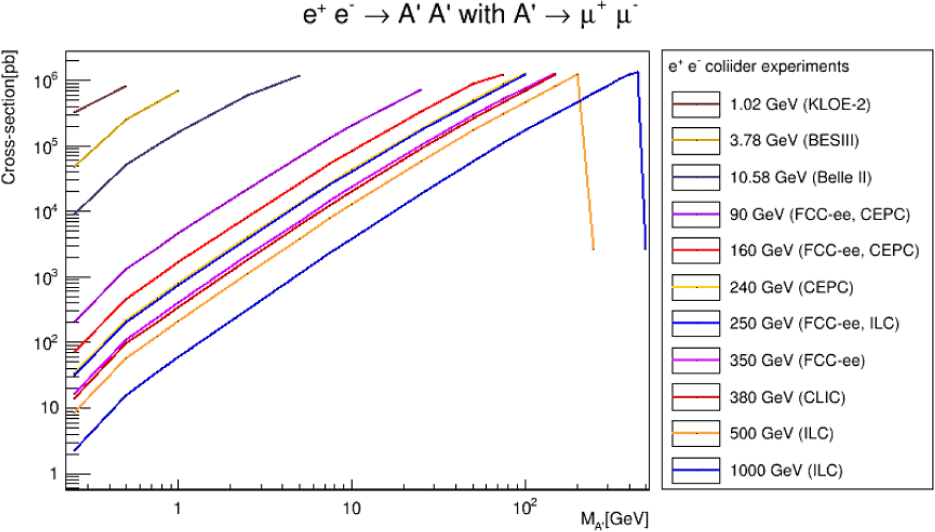
In the previous section, we found dark photon mass with the maximum cross-section for each accelerator. Using these dark photon masses, we performed generation and reconstruction for specific accelerators/detectors. Table 6 shows the specifications of event generation and reconstruction. The KISTI-5 supercomputer was used for event generation, while a local machine, Scientific Linux OS with 32 cores, was used for detector simulation, reconstruction, and fitting. Detector simulation was performed by applying Delphes cards (Delphes 2014) for each detector. Next, the physical quantities for dark photons were reconstructed using C++ and ROOT (Brun & Rademakers 1997). Finally, the invariant masses of the dark photons were fitted using RooFit (Verkerke & Kirkby 2003). Table 7 lists the reconstruction configuration of each accelerator/detector. Table 8 shows parameters of Delphes for each accelerator/detector. The difference of parameters among Delphes results in difference of detector efficiency (Delphes 2014).
Accelerator/detector | [GeV] | mA′ [GeV] | Delphes card (.tcl) (Delphes 2014) |
---|---|---|---|
CEPC/CEPC | 91 (e+: 45.5, e−: 45.5) | 25 | Delphes_card_CEPC |
160 (e+: 80, e−: 80) | 75 | ||
240 (e+: 120, e−: 120) | 100 | ||
FCC-ee/IDEA | 91 (e+: 45.5, e−: 45.5) | 25 | Delphes_card_IDEA |
160 (e+: 80, e−: 80) | 75 | ||
250 (e+: 125, e−: 125) | 100 | ||
350 (e+: 175, e−: 175) | 150 | ||
ILC/ILD | 250 (e+: 125, e−: 125) | 100 | Delphes_card_ILD |
500 (e+: 250, e−: 250) | 200 | ||
1,000 (e+: 500, e−: 500) | 450 |
Accelerator/detector | CEPC/CEPC (Delphes 2014) | FCC-ee/IDEA (Delphes 2014) | ILC/ILD (Delphes 2014) |
---|---|---|---|
Magnetic field (B) | |||
Radius [m] | 1.81 | 2.25 | 1.8 |
Half-length [m] | 2.35 | 2.5 | 2.4 |
B [T] | 3.5 | 2.0 | 3.5 |
Muon | |||
Tracking condition of η | |η| ≤ 3.0 | |η| ≤ 3.0 | |η| ≤ 2.4 |
Tracking condition of pT [GeV] | > 0.1 | - | > 0.1 |
Tracking condition of E [GeV] | - | - | - |
Fig. 5 shows dark photon physical quantities at GeV (CEPC/CEPC), GeV (FCC-ee/IDEA) and at GeV (ILC/ILD). Each figure shows physical quantities of the invariant mass of two dark photons , the invariant mass of a dark photon , and the transverse momentum of a dark photon in the decay mode of e+e− → A′A′ (Park et al. 2020). To find the muon pair that originates from the associated dark photon, we selected dark photons by choosing the least dark photon mass difference (Park et al. 2021). It reduces the random combinatorial background of μ+μ−. The expected number of events has been obtained by fitting and , with double Gaussian function for both decay mode of e+e− → A′A′.
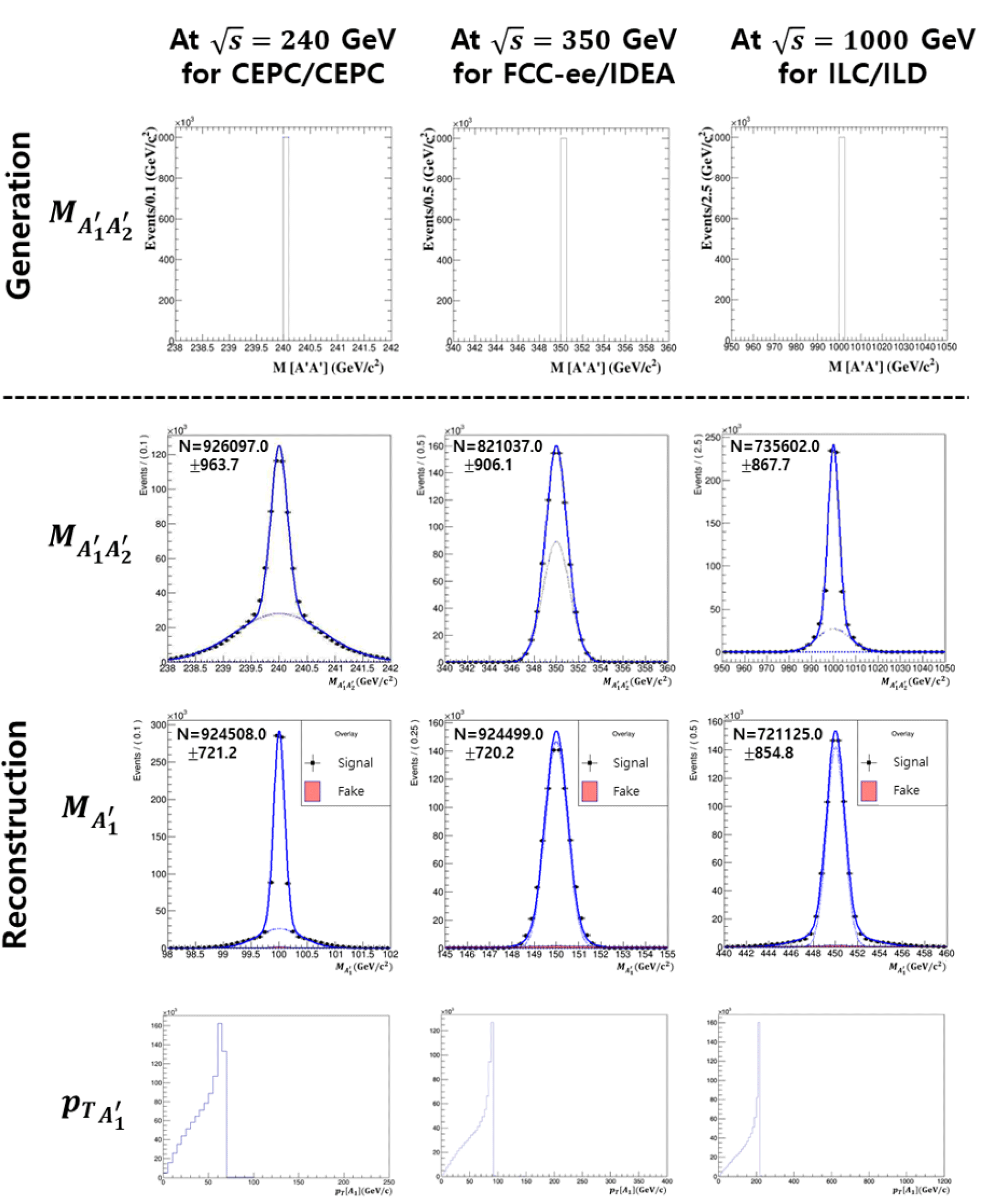
The results show that the CEPC had the highest detector acceptance among the accelerators. Table 9 summarizes the detector efficiencies of the accelerators/detectors. The difference in the detector efficiencies was attributed to the different Delphes parameters. Fig. 6 shows the detector efficiencies of each accelerator/detector with decay mode of e+e− → A′A′, respectively. These results show sensitivities of the physical potentials of the different electron-positron colliders.
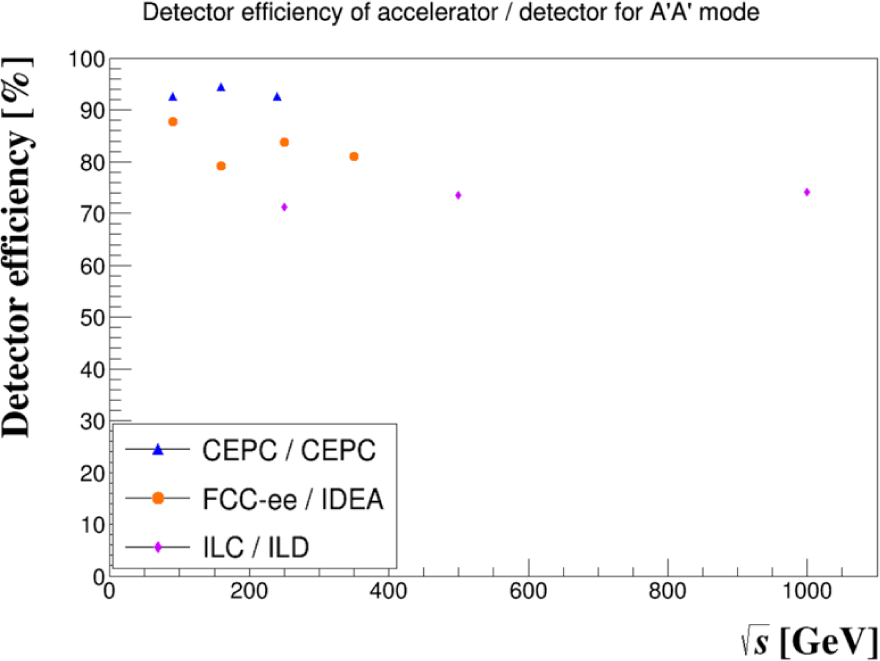
5. SUMMARY
We studied the double dark photon modes of future electron-positron accelerators/detectors. The signal mode was e+e− → A′A′, where A′ decaying into a muon pair. We studied sensitivities of double dark photon modes at CEPC/CEPC, FCC-ee/IDEA, and ILC/ILD using the parameterized response of the detector simulation of Delphes. For the generation study, we determined the dark photon mass dependency of the cross-section. We found the dark photon mass at which the cross-section was the highest for each experiment, and these dark photon masses were adapted for reconstruction. In the reconstruction study, we determined the expected number of signal events. Dark photons were selected by selecting the least dark photon mass difference to reduce the random combinatorial background. Finally, the detector efficiencies of each accelerator and detector were determined using Delphes. These results show sensitivities of the physical potentials of the different electron-positron colliders. The results can facilitate in identifying double dark photon mode in future electron-positron accelerators and detectors.