1. INTRODUCTION
The Korean heliospheric community, led by the Korea Astronomy and Space Science Institute (KASI) and supported by the Korean government, is currently engaged in a feasibility study in collaboration with a National Aeronautics and Space Administration (NASA) group to explore the possibility of siting a spacecraft at the Sun-Earth Lagrange point L4. This initiative involves a spacecraft equipped with various remote-sensing and in situ instruments, as outlined in the studies by Cho et al. (2023). The strategic placement of the spacecraft at L4, which is located 60° ahead of Earth in orbit, offers distinct advantages, particularly when integrated with other space observatories positioned at L1 and L5 (Posner et al. 2021).
At Lagrange Point L1, NASA plans to deploy the Space Weather Follow On Lagrange 1 (SWFO-L1), slated for launch as a rideshare with NASA’s Interstellar Mapping and Acceleration Probe (IMAP) mission in 2025 (https://www.nesdis.noaa.gov/next-generation/space-weather/swfo-instruments). Additionally, the European Space Agency (ESA) is contemplating the siting of another spacecraft, named Vigil, at L5. Vigil is designed to offer advanced warning of solar storms, providing valuable time for enhanced space weather prediction efforts (https://www.esa.int/Space_Safety/Vigil; https://space.oscar.wmo.int/satellites/view/vigil_l5).
As introduced in Cho et al. (2023), in situ measurements of the L4 mission will be conducted by means of various instruments, including a solar wind plasma analyzer, high-energy particle detector, fluxgate and search coil magnetometers, radiation monitor, radio/wave detector, and dust detector. By combining in situ observational data from these instruments with remote sensing observations, as well as data from L1 and L5, we anticipate significant scientific advancements and breakthroughs in various key research areas.
The aim of this paper is to provide a comprehensive overview of major science topics, primarily leveraging the anticipated in situ observations at L4, complemented by data from L1 and L5. The objective is to assist and guide the ongoing feasibility study conducted by KASI. Recognizing that the launch of the L4 spacecraft is anticipated to be 10 years or later, we have chosen to prioritize science topics that necessitate long-term research efforts. We endeavor to actively identify and organize research topics, primarily led by researchers in the Korean heliospheric community, alongside their international colleagues. However, we acknowledge the possibility of unintentionally overlooking emerging research topics in the future.
2. LONG-TERM SCIENCE ISSUES
Conducting in situ observations at L4 is expected to enhance our understanding of large-scale phenomena and structures in the solar wind, particularly when integrated with observations at L1 and L5. Here, we have chosen three specific subjects, which stand to gain valuable insights from observations at multiple Lagrangian points.
Coronal mass ejections (CMEs) are the primary cause of geomagnetic storms, which has made them a consistent and enduring focus of significant research efforts. As they propagate through the heliosphere, they continually interact with the ambient solar wind, leading to their speeds generally decelerating or occasionally accelerating (e.g., Vršnak & Gopalswamy 2002; Vršnak 2006). This interaction can impede our ability to predict the arrival time of CMEs and associated shocks at Earth (e.g., Kim et al. 2007). By tracking CME propagations and eventually directly confirming the interplanetary coronal mass ejection (ICME) arrival at 1 AU, we can obtain more precise insights into CME acceleration and deceleration. Coordinated observations at L1 and L4 can certainly enhance progress toward this scientific goal. Fig. 1 illustrates remote sensing observations at L1 and in situ observations at L4 for a CME event. Such coordinated observations are also crucial for understanding other significant CME-related issues, including structural deformation after CME-CME interactions (e.g., Scolini et al. 2020) and the extended sheath and shrunken magnetic clouds following interactions with the solar wind.
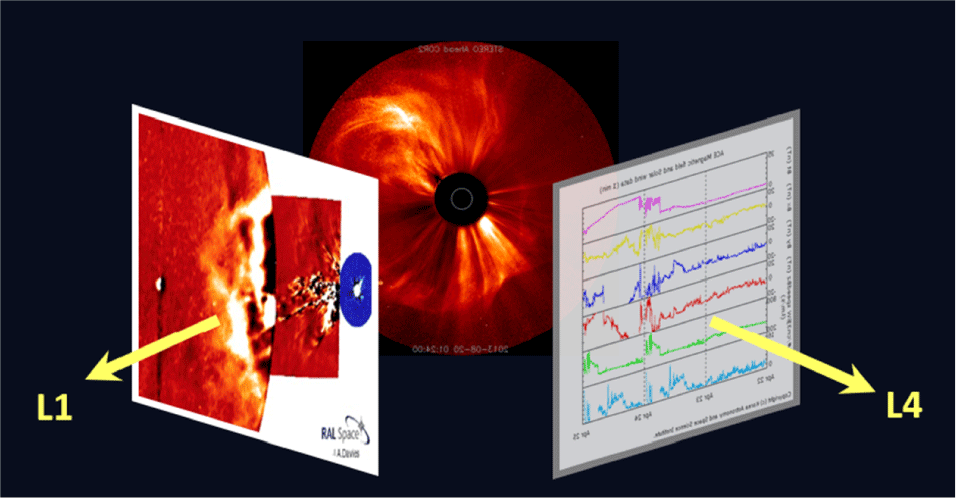
CMEs, and eventually ICMEs, undergo continuous evolution in both temporal and spatial dimensions (Kim et al. 2013). Despite long-term continuous observations at L1, single-point observations have limitations in fully understanding the structure and evolution of ICMEs. It is clearly desirable to have multiple-point observations with proper alignment of the observing spacecraft (see examples in Fig. 2). Placing a spacecraft at L4 and coordinating with L1 and L5 should fulfill such scientific goals to some extent. As inferred in Fig. 2, a radial alignment of multiple spacecraft (green) is useful for understanding the temporal evolution of CME rotations, deflections, etc., while a longitudinal alignment of spacecraft (red), such as at the three Lagrange points, can help clarify the spatial structure of ICMEs, thereby increasing the reliability of magnetic cloud modeling (further discussed below). Without a specific alignment (black), distinguishing between the radial and longitudinal dependencies of ICME properties is challenging.
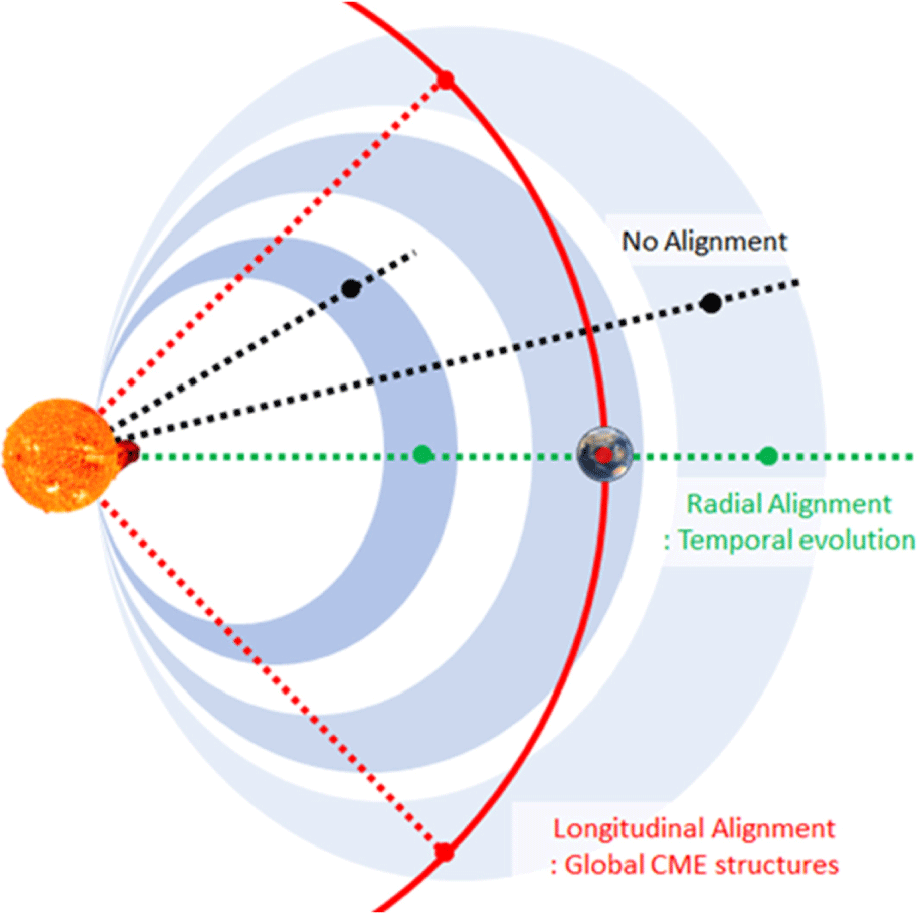
Multipoint observations should offer an integrated view of the cross-sectional structure of ICMEs, a precision not achievable by a single spacecraft, as demonstrated in Gopalswamy (2006) and Kim et al. (2013). This approach will facilitate the determination of the global magnetic flux rope structure of ICMEs in a more accurate manner. Ultimately, it will contribute to predicting the precise ICME structure that interacts with Earth’s magnetosphere, thereby enhancing the reliability of space weather predictions.
ICMEs often exhibit a distinct large-scale structure in the form of a magnetic flux rope known as a magnetic cloud. A similar flux rope structure on a mesoscale (Viall et al. 2021), considerably smaller than the scale of magnetic clouds but still larger than the kinetic scale, has been regularly observed at nearly all heliospheric distances, irrespective of the sunspot cycle phase (e.g., Chen & Hu 2020; Choi et al. 2021; Chen et al. 2023). These structures are referred to as small-scale magnetic flux ropes (SMFRs). The spatial scale of SMFRs is typically one-tenth that of magnetic clouds when observed at 1 au. In contrast to the extensive history of typical magnetic cloud research, SMFR research has a relatively shorter history, and our understanding of these clouds is still limited, necessitating sustained and intensive research. Currently, the origin and specific structure of such SMFRs are active research subjects in the heliospheric science community, in which both solar origins due to small ejecta or reconnections near helmet streamer tips and interplanetary origins due to reconnections and turbulence are considered (e.g., Rouillard et al. 2011; Zheng & Hu 2018; Choi et al. 2022, 2024). Moreover, SMFRs are closely associated with switchbacks which are characterized by local short-term deflections of the magnetic field (e.g., Jagarlamudi et al. 2023). These switchbacks have been frequently observed by the Parker Solar Probe in close proximity to the Sun and, at other outer heliospheric distances, by spacecraft such as Helios, WIND and Ulysses (e.g., Horbury et al. 2018). Investigating the precise relationship between SMFRs and switchbacks is a subject of intense research (e.g., Drake et al. 2021).
Given the high detection rate inferred from numerous single spacecraft observations, there is no reason to preclude the possibility of the near-simultaneous existence of more than one SMFR at different locations. Comparing such SMFRs at two or three Lagrange points presents a compelling avenue for advanced research. SMFRs are typically well distinguished from higher-density background solar wind and are often referred to as blobs (as shown in Fig. 3). Spacecraft at different Lagrange points can encounter SMFRs in various ways, as depicted in Fig. 3, which illustrates a set of possible scenarios for satellite crossings through SMFRs and the corresponding background (Sanchez-Diaz et al. 2019).
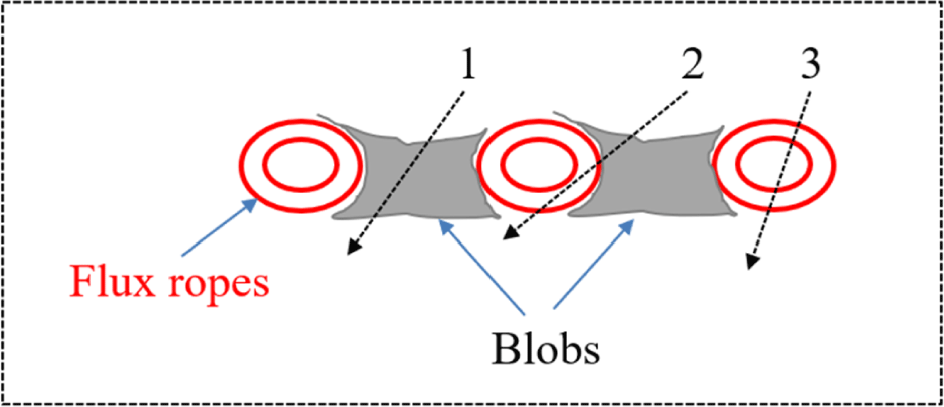
Recent investigations, drawing on comparisons between observations at different heliospheric distances, such as those from the Parker Solar Probe in close proximity to the Sun and those at 1 AU by WIND, reveal that while large-scale heliospheric current sheets (HCS; discussed below) remain largely consistent, small-scale structures such as flux ropes and blobs exhibit significant differences (Szabo et al. 2020). This suggests a noteworthy evolution in the inner heliosphere that is potentially influenced by the longitude. A challenging yet promising endeavor would involve comparing small-scale structures, such as SMFRs, among different longitudinal points (the three Lagrange points), coupled with a radial comparison, to comprehensively understand the evolution of SMFRs.
The HCSs are an interplanetary extension of the solar neutral line (Schulz 1973). HCSs can be warped (Jokipii & Thomas 1981), and the degree of warping depends on the solar cycle phase. Consequently, the likelihood of a spacecraft encountering HCSs at high latitudes increases when warping is significant, a phenomenon typically observed near the solar cycle maximum, as illustrated in Fig. 4 (Hoeksema et al. 1983).
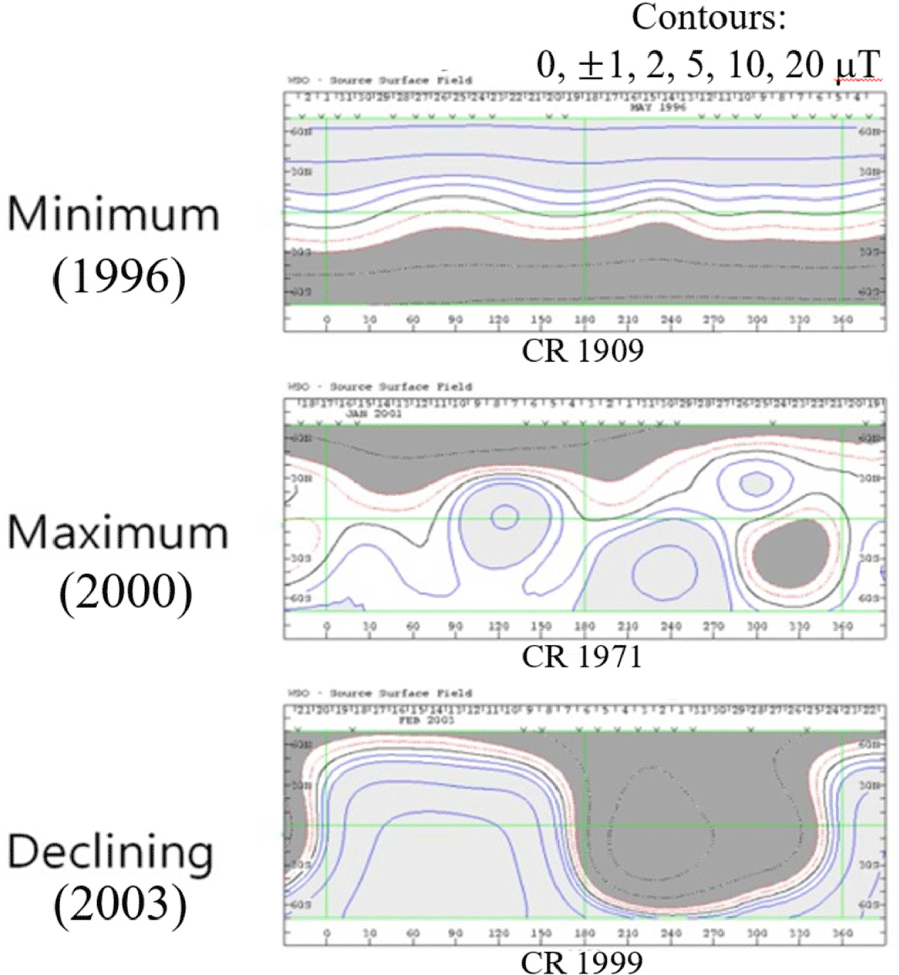
Moreover, the HCS structure is intricate due to variations in the orientation (or local tilt angle), a broad range of thickness [from ~100s km to ~106 km (~7 × 10–3 au)], the potential existence of multiple current sheets, and the distortion and reformation caused by propagating ICMEs (Jokipii & Thomas 1981; Winterhalter et al. 1994; Lepping et al. 1996; Smith 2001; Blanco et al. 2008; Neugebauer 2008; Liou & Wu 2021). The radial dependence of the HCS has been previously investigated, including examinations at distances very close to the Sun based on Parker Solar Probe observations (Lavraud et al. 2020; Szabo et al. 2020) and at far distances in the inner heliosheath (Burlaga et al. 2018; Choi et al. 2023). However, there is a notable scarcity of research on the longitudinal dependence of HCSs, and our current understanding of this aspect is relatively limited. As depicted in Fig. 5, observations from multiple Lagrange points may reveal diverse structures of the HCS, if identified, thereby contributing to a more comprehensive understanding of these complex issues.
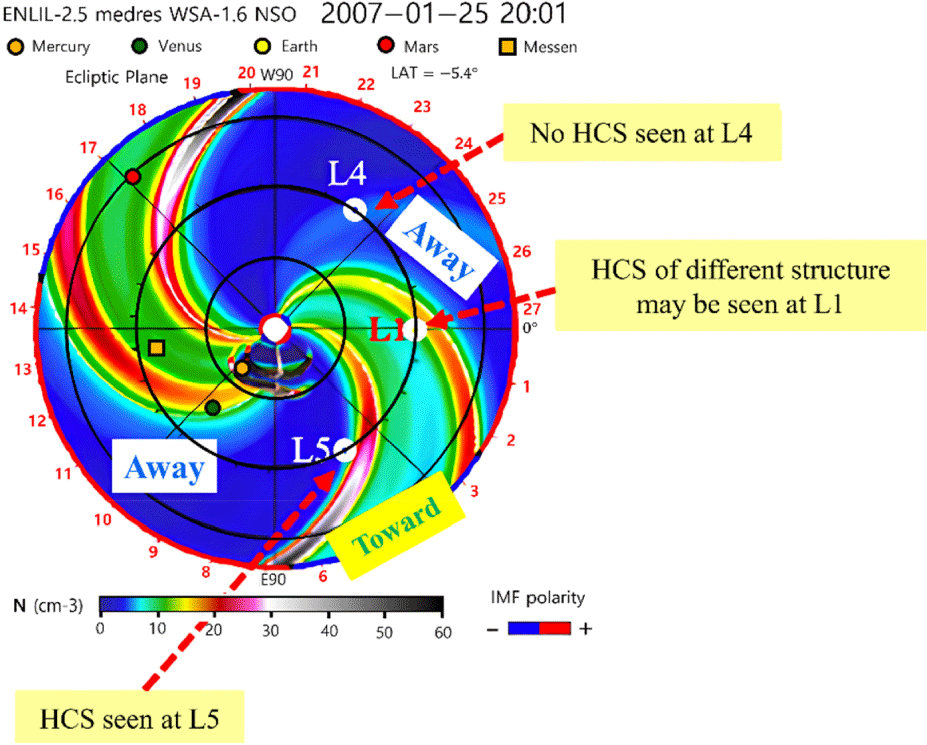
Suprathermal and energetic particles constitute crucial elements in the L4 mission. The forthcoming spacecraft is equipped with in situ instruments dedicated to the study of these particles, facilitating a diverse range of research. Along with remote sensing at L4, coordinated observations at multiple Lagrangian points will significantly amplify the benefits derived from investigating these particles.
Suprathermal isotropic halos and field-aligned strahl electrons constitute approximately 4%–10% of the solar wind electron population. Notably, strahl beams are instrumental in determining magnetic field geometry, indicating whether the field is open, closed, or disconnected (Owens & Forsyth 2013). These beams are most effectively observed/identified at typically 100s eV and are often broad, possibly due to interactions with plasma waves such as whistler waves (Pagel et al. 2007; Cattell & Vo 2021). While bi-directional beams generally suggest a closed field line geometry, complications can arise due to the presence of local field enhancements, primarily associated with co-rotating interaction regions (CIRs) and interplanetary shocks ahead of ICMEs (Choi et al. 2021). Fig. 6 illustrates how such situations can occur, and diverse situations may be encountered through observations at different Lagrange points.
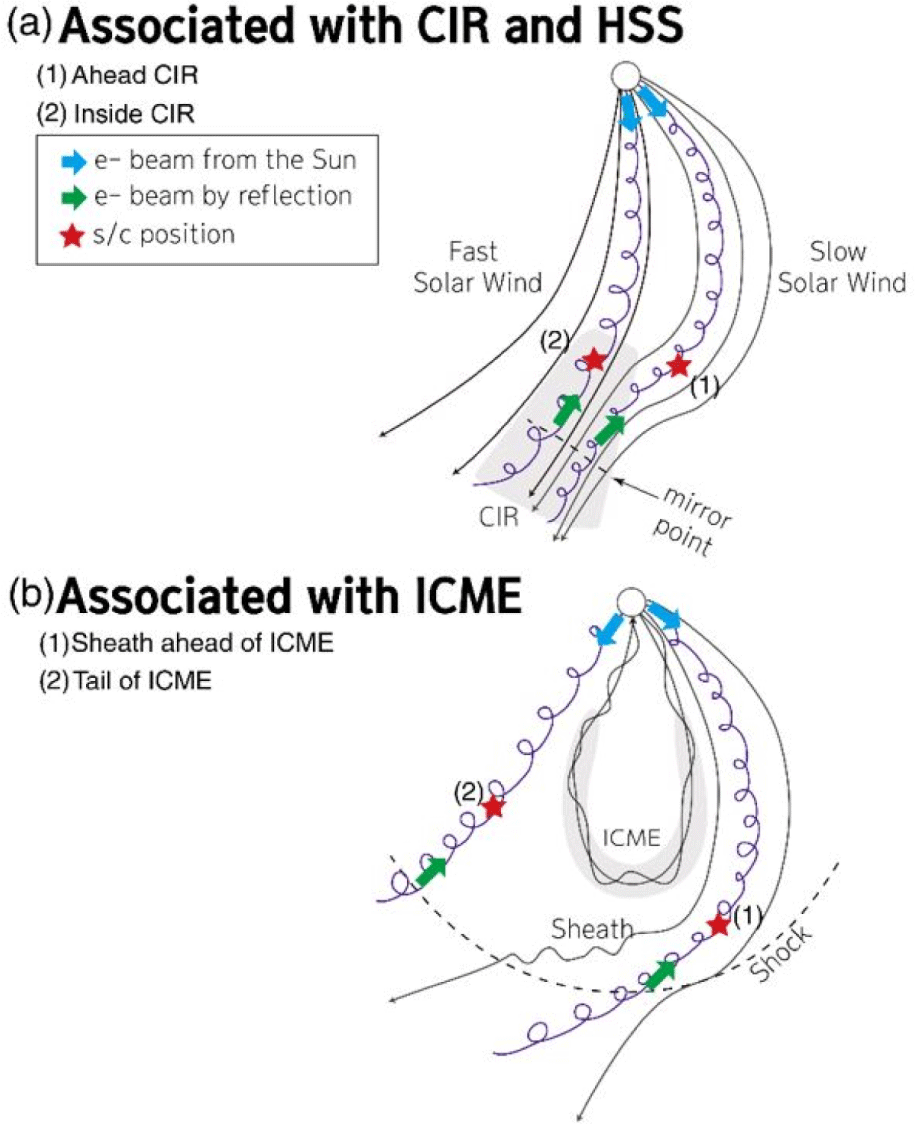
The electron strahl beams can be used to determine the true sector boundary (TSB) between heliospheric magnetic polarity changes, signifying that the strahl beam direction switches from parallel propagation to antiparallel propagation (or vice versa) along the magnetic field lines in each hemisphere (Lavraud et al. 2020). While this principle suggests the co-location with the HCSs, in reality, they are not necessarily consistent with each other. Since the HCS can be defined from the magnetic field polarity reversal, more than one current could exist within a broader HCS layer. Fig. 7 illustrates a possible scenario where the interchange reconnection between an initially closed loop and an open field line creates the HCS, while the true sector boundary defined by the strahl beam directions is located somewhat differently. Such interchange reconnection-generated loops are transient and evolve over time, and spacecraft at different positions, such as L4 and the other Lagrange points, may encounter different structures of the HCS and true sector boundary. Hence, a meticulous analysis incorporating both suprathermal electron and magnetic field data is essential for obtaining a clear and unambiguous distinction between the TSB and the HCSs.
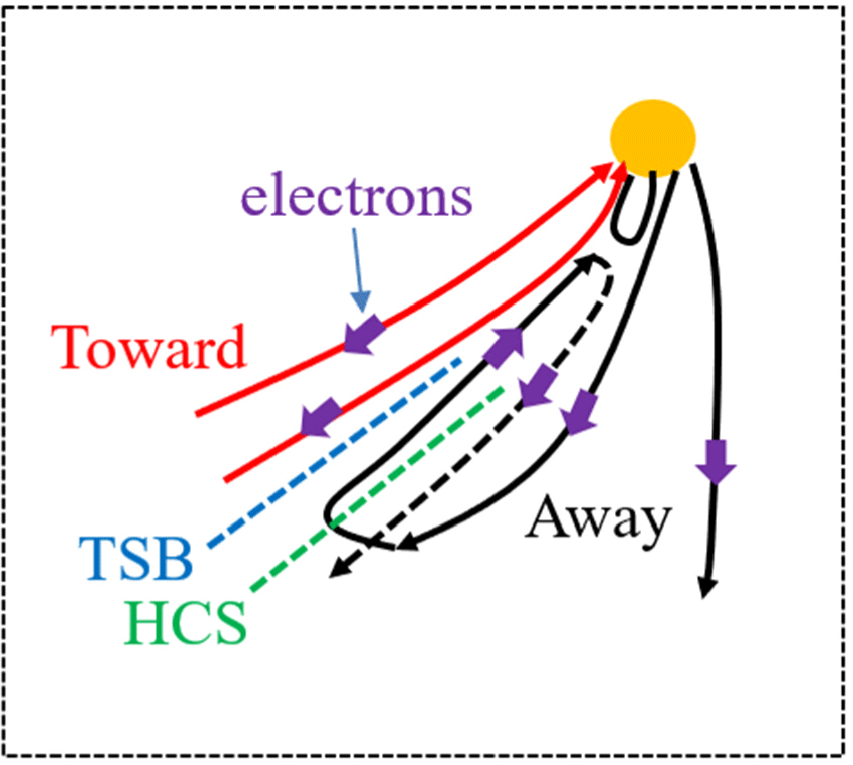
Intense solar energetic particles (SEPs), as highlighted by Desai & Giacalone (2016), can potentially cause substantial radiation harm to humans during space exploration, particularly as we approach the era of lunar and Mars exploration (Cucinotta et al. 2010). The risk of radiation exposure to astronauts in space primarily stems from SEP events involving protons of approximately 50 MeV. The significance of the L4 mission lies in its pivotal role in predicting and forecasting these hazardous radiation risks, extending beyond mere academic interest and directly impacting the safety of astronauts.
While CMEs propagate in a near-radial fashion from the Sun to 1 AU and beyond, the movement of energetic particles is generally guided by magnetic fields within the heliosphere. Notably, at 1 AU from the Sun, particularly near the ecliptic plane, SEPs are magnetically linked to flares/CMEs originating ~60° west of the Sun (Posner et al. 2021), an area ideally observable by the L4 mission. Additionally, Richardson et al. (2014) documented that approximately 30% of the most hazardous SEP events during the two-STEREO era originated behind the western solar limb relative to a 1 AU observer, including Earth. However, there is a scarcity of observations of flares occurring behind the western solar limb. Observations from the L4 mission will provide crucial data for refining models and predictions (further discussed below) related to space weather, particularly SEPs, which are vital for safeguarding human activities and assets in space.
Conventionally, two kinds of SEPs are considered (e.g., Reames 2020). Impulsive events are those caused by flares associated with impulsive Hα and X-ray flares or jets for short durations (~several hrs.). They mostly occur in the western region, which is magnetically well connected to Earth. These events are 3He-rich and frequently accompanied by type III radio bursts, the ideal tracer of energetic electron escape from the magnetic reconnection site. Particle acceleration in impulsive events starts from lower energy levels [as shown in Fig. 8(a)], progressing rapidly to high energies within a short timescale (Miller et al. 1990; Kim et al. 2014). Gradual events are those caused by CME-driven shocks and are associated with fast CMEs for long durations (~several days), covering a broad longitudinal range. These events are proton-rich and often accompanied by type II radio bursts caused by interplanetary shocks. As shown in Fig. 8(b) (Kim et al. 2014), particle acceleration in gradual events starts from higher energy levels, and low-energy particles arise later as the shock propagates further into the solar wind (Zank et al. 2000).
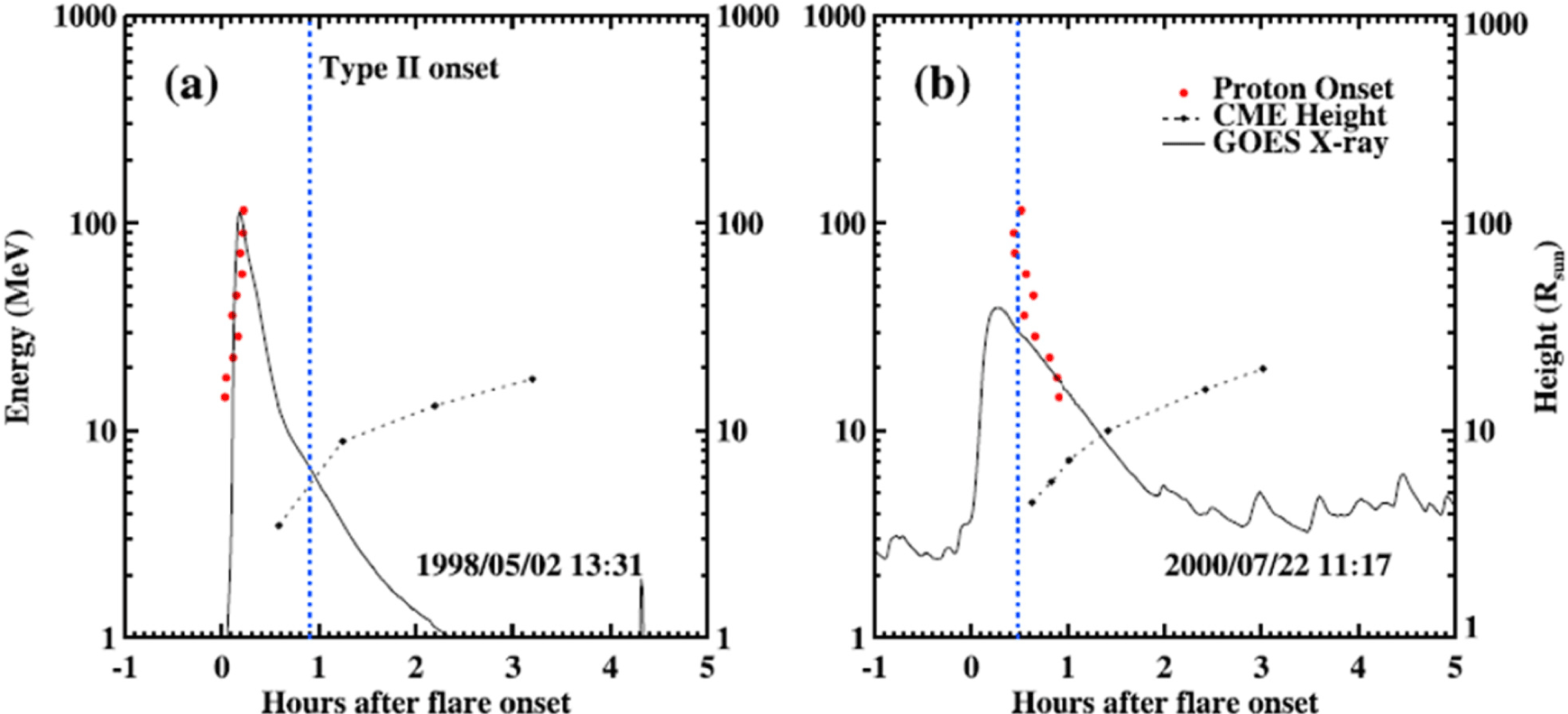
Traditionally, a clear distinction between two types of SEPs is evident in their spatial extent. Impulsive events are typically observed over a narrow range of heliographic longitudes due to the spatially compact acceleration region. In contrast, gradual events are typically observed over a broader range in longitude, as the injection of accelerated particles occurs on magnetic field lines spanning a wider longitudinal range as the shock intersects these lines near the Sun. Exceptional cases exist, indicating that individual impulsive SEP events were observed at multiple spacecraft situated across a longitudinally broad range (Reames et al. 1991; Wiedenbeck et al. 2010, 2013). Clearly, understanding the longitudinal dependence of flare-accelerated particles requires multiple spacecraft observations. Additionally, time delays in SEP onset and peak intensity increase with an increasing connection angle between the solar event and the foot points of the magnetic field lines passing through the observing spacecraft (Richardson et al. 2014). Therefore, in situ observations at multiple Lagrange points, including L4, should play a vital role in resolving all these issues.
The L4 mission can extend its benefits to kinetic-scale sciences, including kinetic plasma waves and instabilities that are locally excited but may propagate on a large scale. SEPs serve as the origin of some solar radio bursts, acting as a proxy for early warnings of energetic events originating in proximity to the Sun. The intricate connection between solar radio bursts and nonlinear plasma physics at the kinetic scale further underscores the mission’s significance.
A series of studies has reported widespread observations of enhanced magnetic fluctuations near proton/electron cyclotron frequencies in the inner heliosphere (Jian et al. 2009; Breneman et al. 2010; Lacombe et al. 2014; Boardsen et al. 2015; Gary et al. 2016; Stansby et al. 2016; Bale et al. 2019; Zhao et al. 2019; Tong et al. 2019; Agapitov et al. 2020; Bowen et al. 2020; Cattell et al. 2020; Verniero et al. 2020; Liu et al. 2023). These electromagnetic waves at the ion/electron scale include Alfven ion cyclotron, fast magnetosonic, and whistler waves, and they are believed to play a crucial role in heating, accelerating, scattering coronal and solar wind plasma particles and regulating heat flux in solar wind through wave‒particle resonant interactions (Pagel et al. 2007; Cattell & Vo 2021; Bowen et al. 2022; Squire et al. 2022; Raouafi et al. 2023). While many issues concerning the origin of such kinetic-scale waves are still debated, recent observations suggest that these waves could be locally generated in an interplanetary medium through plasma kinetic instabilities (Gary et al. 2016; Liu et al. 2023). Despite the well-known fact that velocity space micro-instabilities arise when the plasma deviates far from thermal equilibrium, the underlying physical mechanisms giving rise to the non-thermal features of particle velocity distributions observed in the inner heliosphere remain unclear.
Crucially, multi-scale coupling between global solar wind expansion and local kinetic processes governs the dynamic and thermodynamic evolution of solar wind plasma (Verscharen et al. 2019; Seough et al. 2023). These complex interactions can be traced through both in situ measurements of local plasma properties (for small-scale processes) and remote sensing observations of the origin of global structures (for large-scale processes). These fluctuations may result in a concomitant variation in the particle velocity distribution, consequently generating plasma waves at kinetic scales. In-situ observation data from L4, along with data from other Lagrangian points, should enhance our comparative understanding of kinetic nature among different locations. This approach is valuable for clarifying the aspects in which kinetic physics differs at various longitudinal locations in comparison with differences in the radial direction, a topic that has been extensively studied.
The impact of kinetic-scale waves on solar wind particles is notable not only due to their intensive and brief interactions, as elucidated by linear/nonlinear processes (Bowen et al. 2022) but also due to their extended lifespan (Telloni 2021). While most of these waves, induced by kinetic instabilities, are believed to originate in the inner heliosphere (Jian et al. 2009; Jagarlamudi et al. 2021; Liu et al. 2023), observations of the solar wind near Earth consistently reveal the presence of these waves at 1 AU. Additionally, a meticulous analysis of the observed wave properties indicates that certain waves detected at 1 AU are generated and propagate from as close as 0.56 AU (Wei et al. 2016). The remarkable longevity of kinetic waves holds the potential to significantly influence macroscopic solar wind changes. To comprehensively investigate wave propagation on a large scale, collaborative efforts within the heliophysics community are essential. Utilizing data from inner heliospheric missions, including the Parker Solar Probe, Solar Orbiter, and BepiColombo missions, holds the potential to yield valuable insights. Furthermore, the expanded coverage at 1 AU, facilitated by missions such as L4, along with missions at L1 and L5, will enhance opportunities for in-depth conjugate research in this field.
As already alluded to in Section 2.2, it is commonly accepted that type III radio bursts result from energetic electrons associated with impulsive SEP events generated during a solar flare, while type II radio bursts are related to CMEs and gradual SEP events (Reames 2013, 2023). Consequently, the detection of solar radio bursts, encompassing both type II and III bursts (Melrose 2017), is closely tied to the early warning aspects of SEP events. Notably, type III bursts are of particular value as they originate at solar atmospheric altitudes where geo-effective disturbances are most likely to occur. As such, they could serve as a proxy for early warning of energetic events (White 2007). Obviously, having an L4 satellite equipped with a suitable radio antenna to detect these electromagnetic emissions could greatly improve space weather prediction capabilities.
Beyond these pragmatic considerations, the study of solar radio bursts, particularly type III bursts, and, to a certain extent, type II bursts, is important from the perspective of fundamental plasma physics. The basic radio emission mechanism, known as “plasma emission” (Ginzburg & Zheleznyakov 1958; Melrose 1980; MacLean & Labrum 1985), has played the role of a testbed for nonlinear plasma theory, resulting in a recent comprehensive comparative analysis between theoretical predictions and computer simulations (Lee et al. 2019). However, the existing works relate to plasma conditions typical of the interplanetary space characterized by a high ratio of plasma-to-gyrofrequency, i.e., the “unmagnetized plasma” condition. For radio sources close to solar atmospheric altitudes where geo-effective disturbances originate, the assumption of “unmagnetized” plasma may no longer be valid (Morosan et al. 2016). For such a situation, an entirely new nonlinear theory of plasma emission must be formulated. While simulation efforts have recently started to tackle this issue (Lee et al. 2022), addressing this issue through theoretical endeavors constitutes a challenge for the future. An improved plasma emission theory for a magnetized plasma environment could, in turn, help improve the early warning aspects of geo-effective disturbances, for instance, by providing a better model for radiation characteristics such as wave polarization and beaming angle.
The L4 mission has the potential to significantly enhance space weather prediction modeling, covering various aspects, such as CMEs, energetic particles, magnetic flux ropes, space radiation, and solar wind speed and density, over a broad longitudinal range. In situ measurements conducted by the L4 spacecraft will undoubtedly contribute to refining the capabilities of these models.
Multiple-point observations should enhance our ability to reconstruct the propagation and evolution of CME and model particle acceleration processes (e.g., Palmerio et al. 2022). Conventional solar wind propagation models include coronal models (such as the WSA and EUHFORIA-corona), CME models (such as cone, spheromak, torus, and FRi3D), and heliosphere models (such as the Enlil and EUHFORIA-heliosphere models) (Odstrcil 2003; Sheeley 2017; Pomoell & Poedts 2018). Particle acceleration models, such as SEPMOD and PARADISE, have been utilized in the community [see Whitman et al. (2023) for a comprehensive review and related references]. To enhance the performance of such models, higher-resolution input parameters and data assimilation at as many points as possible are needed. As depicted in Figs. 1 and 2 in Wijsen et al. (2019), at the location of the cyan symbol, the cutoff in the particle intensity becomes more gradual due to cross-field diffusion, while at the location of the orange symbol, the effect of cross-field diffusion is to shift the particle onset to an earlier time (right panel). This highlights that the SEP event is a local phenomenon dependent on longitude. Consequently, the accuracy and details of SEP predictions should vary with longitudinal distance from the corresponding CME eruption point. To enhance the performance of such models, continuous multi-point observations combined with appropriate data assimilation methods are indispensable.
Conventionally, interplanetary magnetic flux ropes have been modeled using force-free fitting for low-β (β = the ratio of plasma to magnetic pressure) plasma cases (e.g., Moldwin et al. 1995). The Grad-Shafranov reconstruction is a useful tool for arbitrary β plasma cases (e.g., Zheng et al. 2017), and other researchers have employed magnetic helicity-based techniques to identify flux ropes in solar wind (e.g., Telloni et al. 2012; Zhao et al. 2020). Fig. 9 shows an example where the flux rope is modeled by both force-free fitting and Grad-Shafranov reconstruction since the plasma β is low in this event. Spacecraft at different locations may observe different structures of flux ropes due to evolution during propagation and/or possible longitudinal structures. A comparison of the modeling results for a flux rope event at L4 with those at other positions can certainly enhance our understanding of the spatial and temporal structure of flux ropes. From a technical viewpoint, comparing modeling results among different methods is challenging and requires continuous effort.
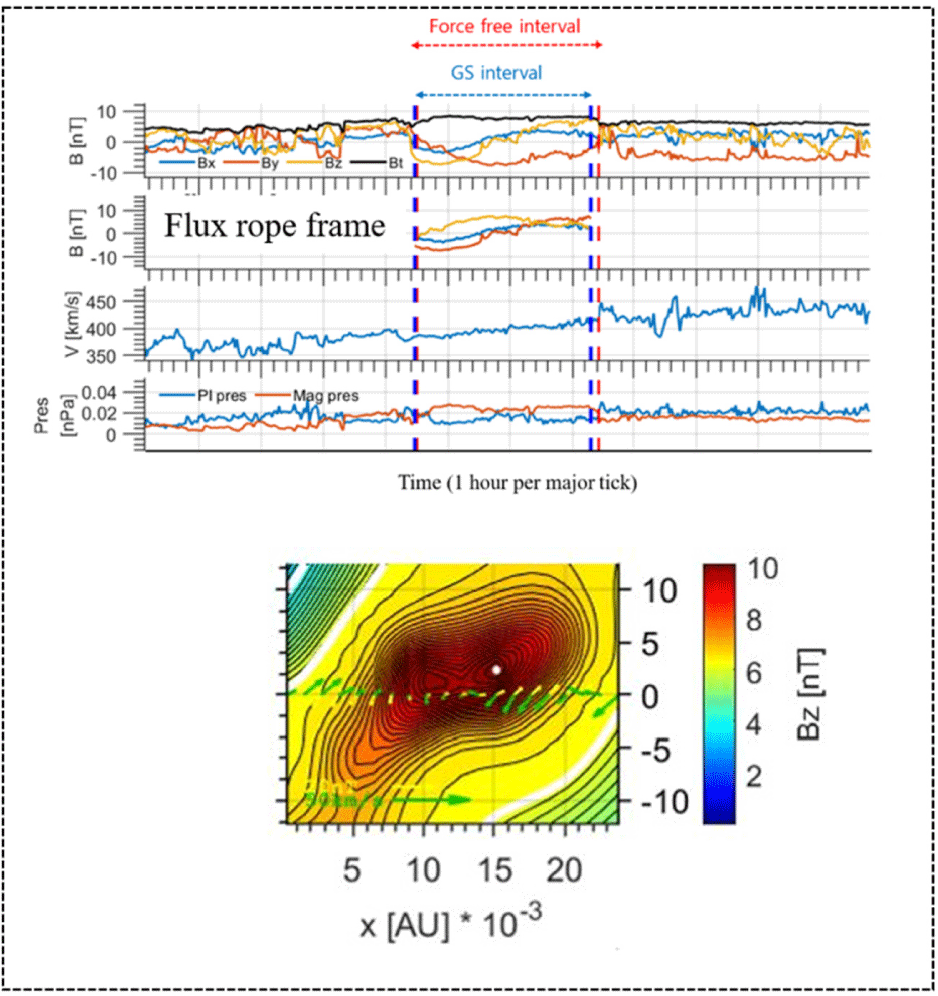
Recently, there has been an increasing necessity for more precise prediction and measurement of aviation radiation in Korea. In the interest of ensuring the radiation safety of our aircrew and passengers, KASI and the Korea Meteorological Administration-National Meteorological Satellite Center (KNMSC) jointly developed their own radiation prediction model, named the Korean Radiation Exposure Assessment Model for the aviation route dose (KREAM) (Hwang et al. 2010, 2014). KREAM is a physics-based aviation radiation dose calculation program designed for altitudes of 0–80 km that incorporates physical models such as GEANT4 and NRLMSIS00 and a cutoff rigidity model (refer to Fig. 10). KREAM utilizes input energy spectra from both galactic cosmic rays (GCRs) and SEPs. SEP information from real-time proton data observed in geosynchronous orbit is integrated into KREAM. Currently, KREAM calculates the effective radiation dose rate to which humans are exposed at aviation altitudes. In the near future, KASI plans to expand the radiation prediction capabilities of KREAM to far higher altitudes, extending into deep space. By incorporating SEP protons at the L4 location, earlier than geosynchronous orbit, the prediction model can work with a longer preceding time, enhancing its usefulness. Furthermore, this extension will cover predictions for low Earth orbit and beyond. The radiation flux/fluence data support the evaluation and prediction of Single Event Effects (SEEs) and total ionization dose (TID) during space missions, including low Earth orbit (Sinclair & Dyer 2013). Calculating the estimated total ionizing dose for a satellite’s lifetime during the manufacturing period is crucial (Hughes & Benedetto 2003). The extended version of KREAM can be used to estimate radiation exposure for both manned and unmanned deep space missions to the Moon and Mars.
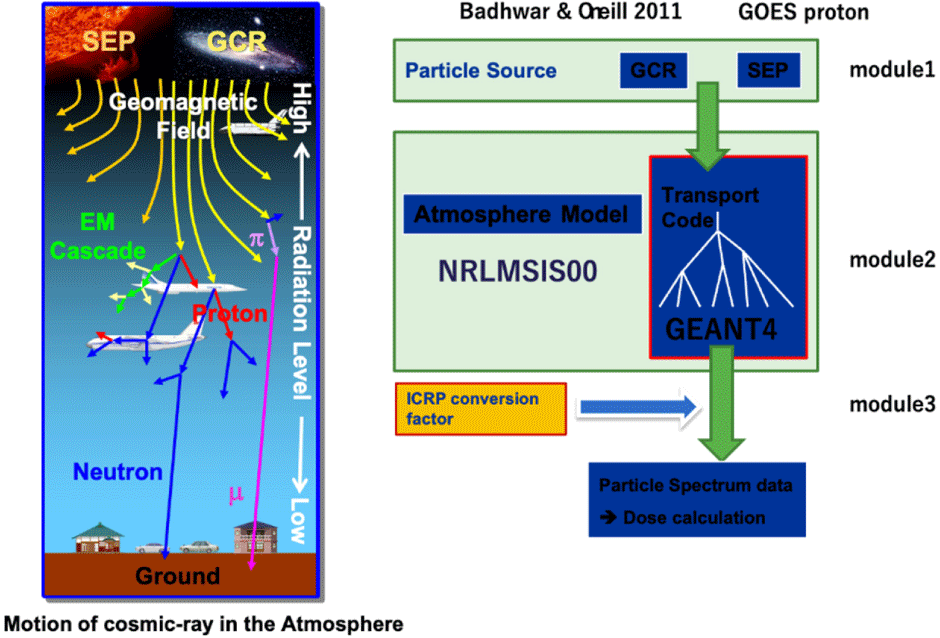
As the solar wind expands outward, traversing the entire heliosphere up to the heliospheric boundary, it undergoes evolution over time due to various factors. One major influence is the charge-exchange interaction with neutral atoms incoming from the local interstellar medium (e.g., Gruntman et al. 2001; Lee et al. 2009). Observations by the Cassini and Interstellar Boundary Explorer (IBEX) spacecraft revealed specific structures (named “belt” and “ribbon”) in energetic neutral atom images, likely produced by charge exchange interactions between solar wind ions and incoming interstellar neutral particles (Funsten et al. 2009; Krimigis et al. 2009; McComas et al. 2009; Schwadron et al. 2011; Dialynas et al. 2013). Another factor is the expansion of ICMEs over a wide longitudinal range and the merging of successive ICMEs (e.g., Burlaga 2015). The details of all such interactions and evolutions depend on the helio-latitude and helio-longitude. The specific states of solar wind speed and dynamic pressure are critical factors in addressing the spatial dependence of these interactions and evolutions (Yoo et al. 2023). Given the limited in situ measurements of solar wind, modeling solar wind parameters becomes essential. One current comprehensive model is based on the reconstruction of solar wind speed and density from interplanetary scintillation (IPS) observations (Sokół et al. 2015; Porowski et al. 2022). Fig. 11 provides an example from IPS predictions of solar wind speed and density in the ecliptic plane compared with OMNI observational data (data for all other latitudes are available, although not shown here). However, a non-negligible discrepancy with the actual OMNI observational data exists, and a contributing factor could be the absence of longitudinal dependence in this model. In situ measurements at L4 and L5 cover 1/3 of the whole longitudinal range, providing a valuable dataset for advancing the solar wind model at 1 AU.
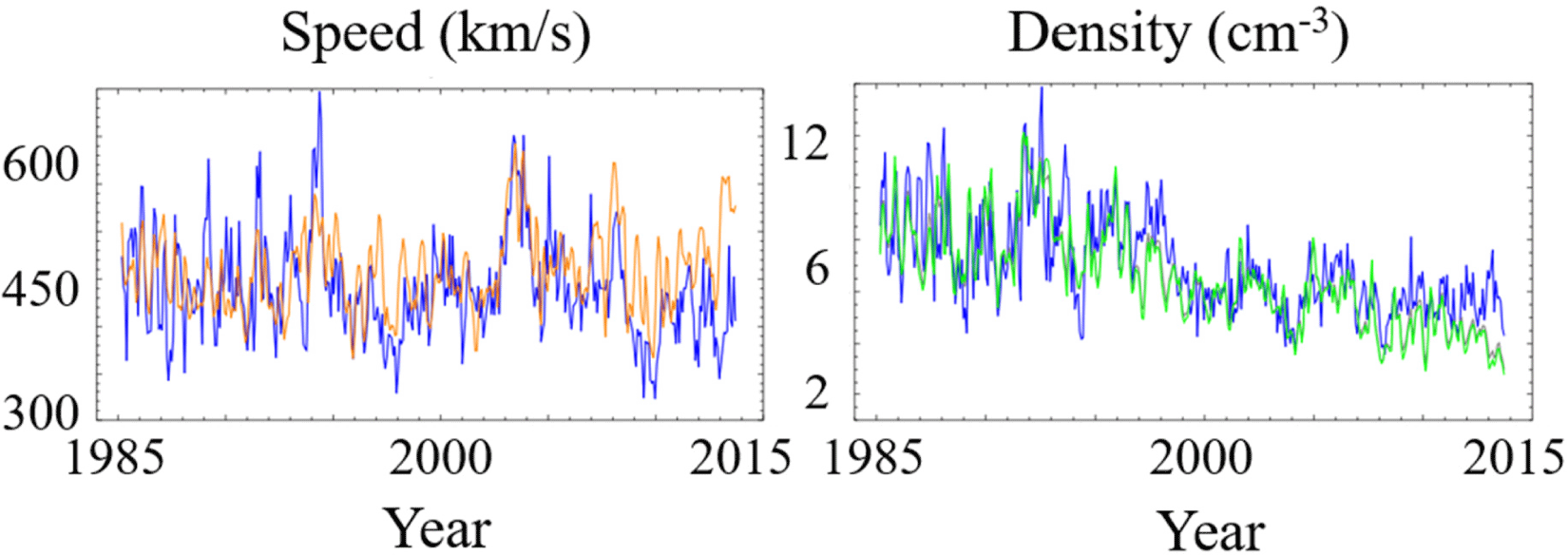
3. SUMMARY and FINAL REMARKS
As of this writing, the Korean heliospheric science community is actively exploring the feasibility of placing a spacecraft at L4, with the expectation of reaching a conclusion in the very near future. This initiative is likely to take the form of international collaboration, with similar missions anticipated at L5 and L1. The L4 spacecraft is envisioned to carry a suite of in situ measurement instruments complemented by remote sensing instruments. In this article, we aim to identify promising science topics that can primarily leverage in situ observations at L4, alongside those at L1 and L5. Anticipating the launch of the L4 mission spacecraft in 10 years or later, our focus has been on long-term science topics. While remaining open to other topics that may emerge in the future, we propose analyzing the following science topics:
-
CME acceleration and deceleration, CME-CME interaction, CME-solar wind interaction, and CME structure (particularly longitudinal structure)
-
Structure and origin of small-scale flux ropes in contrast to typical magnetic clouds
-
Comparison of the structure of HCSs for different Lagrange points and different radial distances
-
Interplanetary field line structure and current sheets: Distinguishing solar magnetic field polarity sector boundaries from HCSs with electron strahl beams
-
Spatial and temporal characteristics of SEPs with a distinction between flare-associated and CME-associated SEPs
-
Kinetic plasma waves and instabilities, their interaction physics, coupling to large-scale structure and solar wind expansion, and their spatial dependence
-
Type III and type II solar radio bursts associated with gradual and impulsive SEPs as early warning indicators of energetic events and testbeds for nonlinear plasma physics
-
Improvement of models for CME evolution and particle acceleration using continuous multi-point in situ observations
-
Advanced modeling of small-scale flux ropes using multi-Lagrange point observations and comparisons among different modeling techniques
-
Advancement of the Korean Radiation Exposure Assessment Model for the aviation route dose (KREAM) model by extending the prediction capability to longer-term prediction times and to low Earth orbit
-
Advanced reconstruction of solar wind speed and density from IPS observations by including a longitudinal dependence
By integrating in-situ observation-based topics with other scientific areas derived from remote sensing observations, we anticipate making a substantial contribution to the progress of the inner heliospheric science field. Moreover, establishing a network for international collaborative research would be advantageous for investigating these research topics. As the Korean heliospheric science community approaches a determination on the feasibility of the L4 mission, this research paper serves as a robust roadmap, laying the foundation for meaningful contributions to the field in the years ahead.