1 INTRODUCTION
Pc1 waves (0.2–5 Hz) have recently been observed at ionospheric altitudes and are known to be attributed to electromagnetic ion cyclotron (EMIC) instability driven by proton temperature anisotropy in the equatorial magnetosphere. Large amplitude (5–30 nT) Pc1 micropulsations were detected near the plasmapause by a low-altitude satellite, magnetic field satellite (MAGSAT), in the ionospheric F region (Iyemori & Hayashi 1989). Ionospheric Pc1 waves are sometimes associated with enhancements in ionospheric electron temperature and low-energy electron flux. The relation between the electron temperature enhancement and large amplitude Pc1 wave injections in the upper ionosphere is investigated using data obtained by the Dynamic Explorer 2 spacecraft (Iyemori et al. 1994). They suggested that the temperature enhancement that accompanies large-amplitude waves with Pc1 pulsation frequencies is caused by the direct acceleration of thermal electrons at low altitudes by the parallel electric field (0.01–0.001 mV/m) of the ioncyclotron waves (kinetic Alfven waves) having oblique wave normals. Magnetic- and electric-field fluctuations in the Pc1 frequency range have been observed by the polar-orbiting Viking satellite (Erlandson et al. 1990). They found that the waves had Poynting vectors directed downward toward Earth, reaching magnitudes between 0.01 and 0.1 erg/cm2s, and the polarization of the waves was found to vary between linear, left-handed, and right-handed as a function of time and latitude. EMIC waves were also observed during the great magnetic storm of April 1993 by an F1 double-probe electric field instrument onboard the Freja satellite (Bräysy et al. 1998). Using ten years of data from the challenging minisatellite payload (CHAMP) satellites, Park et al. (2013) reported that Pc1 magnetic pulsations during solar cycle 23 peak in the early morning sector of the longitude sector of the South Atlantic anomaly (SAA). They also found that neither magnetic activity nor solar wind velocity controls the Pc1 occurrence rate in low earth orbit. Recently, a comprehensive statistical analysis has been performed on the spatial distribution of EMIC waves in low earth orbit by using SWARM satellites from December 2013 to June 2017 (~3.5 years) as a function of magnetic local time (MLT), magnetic latitude, and magnetic longitude (Kim et al. 2018). They found that EMIC waves are detected with peak occurrence in the mid-latitudes, including the subauroral region and dawn sector (3–7 MLT). Linear polarization is oriented primarily in an oblique propagating direction to the background magnetic field.
The source of ionospheric Pc1 waves is known as EMIC waves, created in the equatorial magnetosphere and ducted in the ionosphere. Actually, EMIC waves are considered to play a key role in the precipitation of relativistic electrons into the upper atmosphere through pitch angle diffusion via wave-particle interactions. Therefore, the observations of EMIC waves from these low-altitude satellites can contribute to the study of possible loss mechanisms of energetic particles from the Earth’s radiation belt (Thorne & Kennel 1971; Lyons & Thorne 1972; Jordanova et al. 2008; Miyoshi et al. 2008; Usanova et al. 2014; Shprits et al. 2016). EMIC waves can be observed by ground magnetometers such as the BOH magnetometer (Hwang et al. 2011; Jun et al. 2013). Similar magnetospheric Pi2 pulsations were also observed by Van Allen probe (VAP) satellites (Ghamry 2017). The EMIC at low earth orbit (LEO) might be related to magnetic flux variations in the solar wind (Kim et al. 2017).
Despite the importance of the role of the EMIC in the magnetospheric and ionospheric research, observations of the EMIC wave in LEO have revealed a few clear limitations. First, due to the limitations of low temporal resolution in the technical performance of old magnetometers, it is difficult to detect higher band waves above 1 Hz. Second, the wave signal is easily and periodically contaminated near the aurora region at latitudes greater than about 70°, and the background noise level is much higher than in the outer magnetosphere. Third, it is difficult to discern spatial and temporal variations by using single satellite observations; actually, this is not just the case for LEO observations. To try to overcome such known limitations, a new space mission was recently initiated, using the concept of multi-satellites, named as the Small scale magNetospheric and Ionospheric Plasma Experiments (SNIPE) mission, which is planned to launch in 2020. In this paper, we report the current status of satellite development and preliminary concept of operations and formation flying for the SNIPE mission. We also suggest a possible strategy to overcome the above mentioned limitations in EMIC observations in LEO.
2 DATA
First, we use CHAMP and SWARM data to detect EMIC waves in low earth orbit. The CHAMP satellite was launched in July 2000 into a polar orbit with an inclination angle of about 87°. The initial altitude was about 450 km, decreasing slowly to 250 km at the time of atmospheric re-entry in September 2010. The orbit precessed slowly through local time (LT). It took about 131 days to obtain full LT coverage when considering both up- and down-leg parts. CHAMP carried a fluxgate magnetometer (FGM), measuring the geomagnetic field vectors at a rate of 50 Hz (Park et al. 2013). In this study, however, we use 1-Hz geomagnetic field data, which are available to the public, because we cannot obtain the higher resolution data at this time. To identify Pc1 pulsations, magnetic field data are transformed from north-east-center (NEC) to local field aligned coordinates (FAC) (Rae et al. 2005). We use a Savitzky-Golay smoothing filter (Savitzky & Golay 1964), instead of the mean magnetic field, to define a background magnetic field with a moving window of ± 30 data points (1 min), and a degree of two (secondary polynomial). The fluctuation forms can be obtained by subtracting the filtered magnetic field from the measured magnetic field. The fast Fourier transform (FFT) was conducted with a window of 256 samples (4.3 min), an overlap of ~30 % (~1.3 min). Thus, the wave power spectrum can be obtained with 480 data blocks per day and a 180-s time resolution. Fig. 1(a) shows the one-day wave spectra, which is represented by the covariance signal (Bortnik et al. 2007), observed by the CHAMP satellite on January 1, 2007. The contamination by aurora particles appears repeatedly whenever the spacecraft crosses the auroral regions in both hemispheres. Because of the difficulties prevalent in discerning between temporal perturbed electromagnetic pulsations and fixed contamination due to the aurora, it is difficult to distinguish between EMIC waves and other types of pulsations above the subauroral region. This phenomenon is clearly shown in Fig. 1(b), which is an enlarged plot of Fig. 1(a) during 00:00–02:45 on the same date. The upper panel is the 3-axis magnetic field fluctuation, and the bottom panel is the wave spectra from 0–0.5 Hz. There are some wave features between periodic aurora signals, but they are difficult to resolve as Pc1 pulsations because of the low resolution of the raw data. It is also difficult to analyze wave properties in this lower frequency band by using a single-point observation from a single satellite.
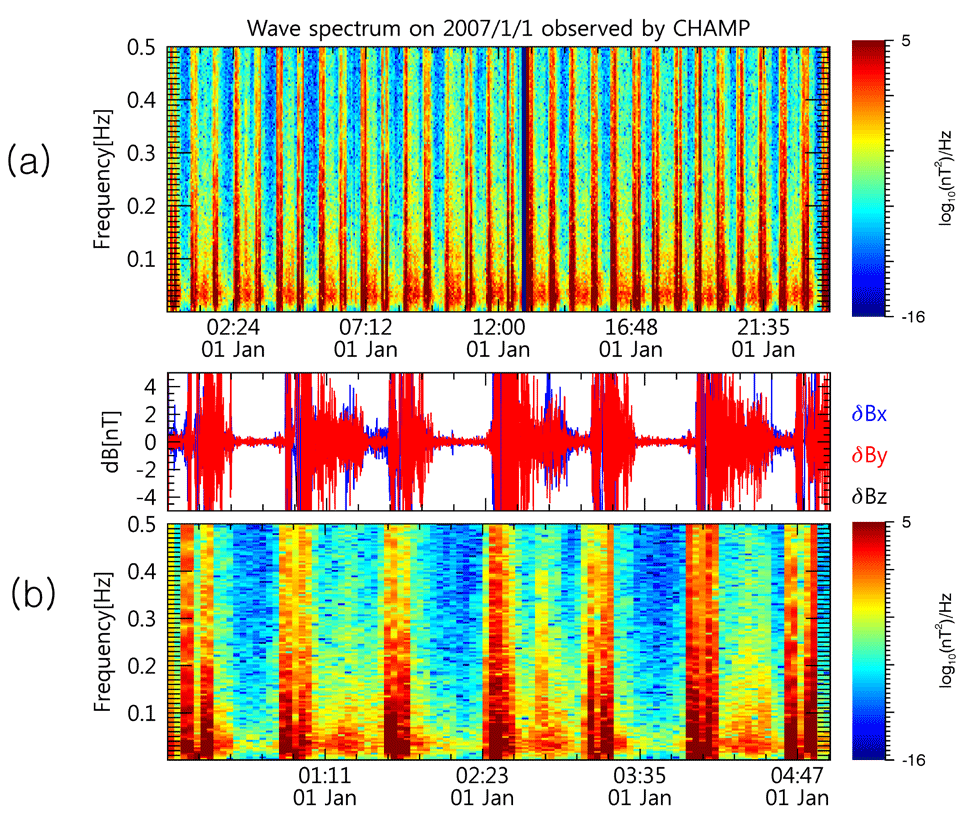
The SWARM satellites were launched on November 22, 2013. The main objective of the SWARM mission is to provide the best observation of the geomagnetic field and its temporal evolution from multi-point measurements using three satellites. The SWARM constellation consists of three satellites (named Alpha, Bravo, and Charlie) placed in two different polar orbits with an inclination of 88°, two flying side by side at an altitude of 450 km and a third at the higher altitude of 530 km. The SWARM mission was deigned to mainly focus on magnetic field measurements, and it measures the threeaxis magnetic field with a high resolution sampling rate of 50 Hz (Nyquist frequency of 25 Hz) and an accuracy of 0.01 nT (Kim et al. 2018). Fig. 2 shows EMIC waves detected by our automatic wave detection algorithm, based on Bortnik et al. (2007), on January 23, 2016, around 06:50 and 09:35, observed by SWARM A. As shown in the middle panel of Figs. 2(a) and 2(b), it is clear that the peak frequency of these waves is about 1.5 Hz, and it is difficult to identify lower band waves at less than 1 Hz because the background noise level is relatively large, which is shown at the bottom panel of Figs. 2(a) and 2(b). Large amplitude noises at the high latitude region (higher than ~70-degree latitude) are also shown by the CHAMP satellite observation, similar to Fig. 1.
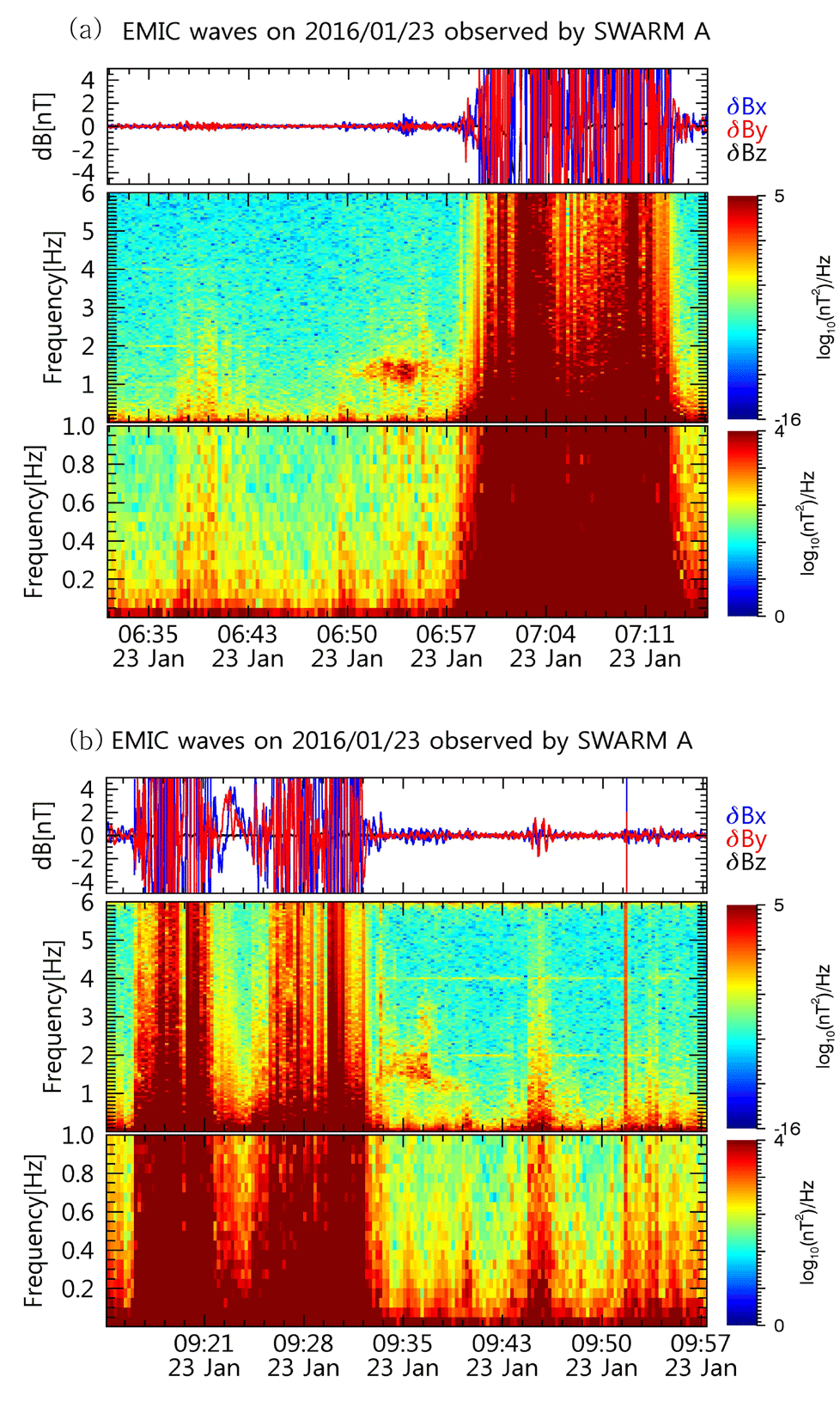
3 SCIENCE TARGET OF SNIPE MISSION
Since 1979, there have been several Pc1 observations in low earth orbit, but their details differ somewhat from case to case. Table 1 shows the previous observations of Pc1 pulsations in low earth orbit by the MAGSAT, DE-2, Viking, Freja, CHAMP, and SWARM satellites. The difficulties in obtaining consistent results among those previous observations might have been due to their geographic location and observation time periods being different from each other and the existence of increased ambiguities in discerning noise and wave signals near the aurora region. It is also critically difficult to separate spatial and temporal features by using single satellite observations. To resolve the spatial and temporal variations of the microscale plasma structures on the topside ionosphere, the Korea Astronomy and Space Science Institute (KASI) recently initiated the SNIPE mission. If we analyze signals from multi-satellites at the same time, we will be able to distinguish the spatial and temporal changes of the waves. SNIPE consists of four 6U nanosatellites (~10 kg) to be launched into a polar orbit at an altitude of ~600 km (TBD) in 2020. Four identical satellites will be deployed in orbit at the same time with a slight time delay and make a precisely controlled formation within a separation distance of ~100 km from each other. The SNIPE mission aims to elucidate the various plasma phenomena with structures of various scales (100 m–10 km) in the topside ionosphere, especially the fine-scale morphology of high-energy electron precipitation, cold plasma density/ temperature, field-aligned currents, and EMIC waves. Particularly, the mission will observe the characteristics of the following plasma phenomena: (1) high-latitude plasma irregularities, such as polar-cap patches; (2) fieldaligned currents in the auroral oval; (3) EMIC waves; (4) precipitation of electrons of hundreds of keV into the atmosphere, such as electron microbursts; (5) subauroral plasma density troughs; and (6) low-latitude plasma irregularities, such as ionospheric blobs and bubbles. We develop a 6U nanosatellite bus system as the basic platform. To achieve those scientific objectives, three types of plasma instruments have been designed: solid state telescope (SST), Langmuir probe (LP), and fluxgate magnetometer (MAG). The dynamic range of the magnetometer will be from -50,000 nT to 50,000 nT and the full width of half maximum of the signal will be 1 nT. The observation sampling rate will be 10 vector/sec. A Langmuir probe of somewhat different type has been developed for NEXTSAT-1 and future space missions (Ryu et al. 2017).
Fig. 3 shows a plot of a preliminary design of formation flying satellites in (a) along-track and (b) cross-track formation. We named the satellites: KASISAT-1, KASISAT-2, KASISAT-3, and KASISAT-4. For convenience, in Fig. 3 we indicated the satellites as A, B, C, and D, respectively. To keep the required formation for at least two geographic locations at the pole and equator, we calculate the total delta V that will be realized by a thruster and, based on those simulation results, we design the proper specifications for the thruster system for the SNIPE mission. Fig. 4 shows a simple diagram showing one of the science targets related to the EMIC observations of the SNIPE mission. We consider observing EMIC waves simultaneously, from both space and ground, and expect to investigate the time evolution features of propagating EMIC waves following the geomagnetic field line from the higher L shell to the lower L shell. We have also developed a method to find a geomagnetic conjunction event between t he different satellites or between space and ground observations. The method to find the conjunction event is as follows:
(1) Set criteria of conjunction:
(2) Read data of satellite positions, satellite footprints, using OMNI, etc.
(3) Examine whether the criteria are met for each satellite for each time step (minute).
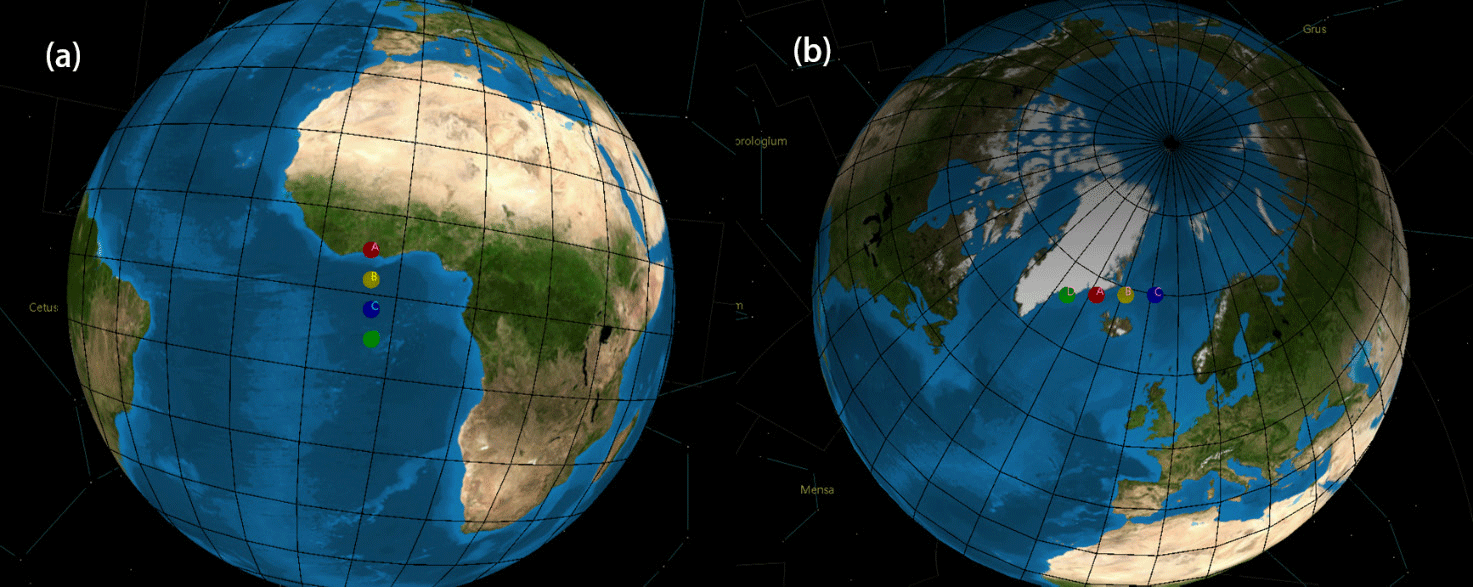
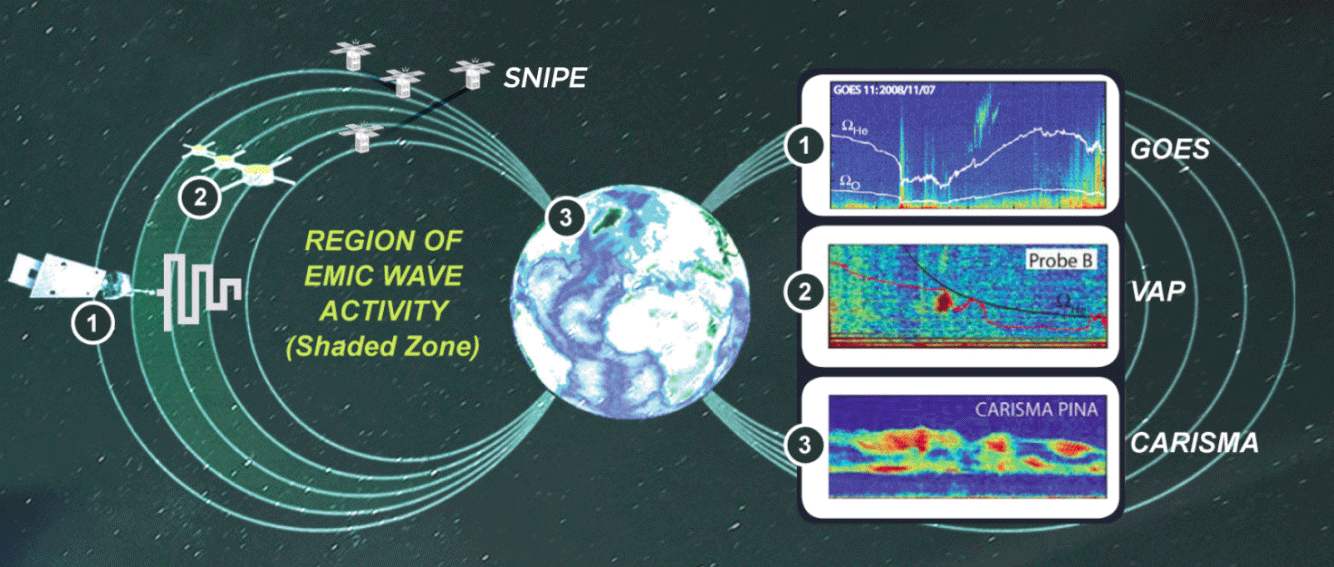
Using the above method, we can find the conjunction event between SNIPE and other satellites such as VAP, exploration of energization and radiation in geospace (ERG), and geostationary operational environmental satellite (GOES), located at geomagnetically different regions in the magnetosphere. As it is known that EMIC waves originate near the equatorial region, we can investigate EMIC waves from their source region in the outer shell to the ionosphere and the ground. Fig. 5 shows a preliminary concept of operation for the SNIPE mission. First, four satellites will be put into their orbits one by one with a slight time difference of a few seconds. After these orbit insertions, the satellites will perform the early operation tasks to reach normal operation mode autonomously. This commissioning phase can take a few weeks or a month. Meanwhile, an in-orbit test (IOT) will be performed to check the status of bus and payload subsystems. For the first two months or so, we maintain the flight through natural drifts without artificial maneuvers. This period is called Science Phase I, as scientific observations will commence during this period. Afterwards, the flight track is artificially controlled to construct an along-track formation by using a thruster (Science Phase II). Thereafter, the propulsion system will be employed again to construct a cross-track formation. After these phases have been completed, the mission is terminated and subsequent operations will be regarded as extended mission periods, with scientific observations continuing during the natural drift without further orbit control.
4 CONCLUSIONS
There have been several observations of Pc1 pulsations in low earth orbit by MAGSAT, DE-2, Viking, Freja, CHAMP, and SWARM A, B, and C satellites. However, there were clear limitations in observing precise Pc1 pulsations in low earth orbit. First, because of the low temporal resolution of the magnetic field instrument, it is difficult to detect higher band waves above 1 Hz. Second, the wave signal is contaminated periodically near the aurora region, and the default background noise level is higher than in the outer magnetosphere. Third, it is hard to discern spatial and temporal variations by using a single-point observation. Actually, those limitations are still difficult to overcome, but we try to resolve at least one limitation through our new mission. Our goal is to discern spatial and temporal variations by using simultaneous observations from multisatellites. In this paper, we report some examples involving EMIC waves observed by CHAMP and SWARM satellites in low earth orbit to explain the difficulties of EMIC observation in LEO. We have also designed the specific systems of the SNIPE mission to find out possible solutions to the unsolved problems in detecting and analyzing various magnetic pulsations in LEO. The SNIPE mission is equipped with scientific payloads that can measure geophysical parameters such as density/temperature of cold ionospheric electrons, energetic (~100 keV) electron flux, and magnetic field vectors. All the payloads will have high temporal resolution (better sampling rates than 10 Hz (TBD)). This mission is planned to launch in 2020.