1 INTRODUCTION
Magnetars are isolated neutron stars powered by strong magnetic fields that are typically greater than 1014 G (Thompson & Duncan 1995) and which emit radiation almost exclusively in the X-ray band. The X-ray emission spectra of magnetars have two main components: thermal emission from the surface and non-thermal radiation in the magnetosphere. Although some magnetars are detected in the optical or infrared band, they are very faint with a magnitude greater than 20. Similar to typical pulsars, magnetars turn with a rotation period (P), and the first derivative () in the range of P = 2 – 12 sec and = 10−13−10−10 sec sec−1. In addition, magnetars often exhibit strong X-ray activities that include brightening in the forms of short bursts, outbursts, and giant flares. These transient behaviors last from milliseconds to years (see Rea et al. 2010; Olausen & Kaspi 2014; Mereghetti et al. 2015 for review).
The first magnetar was discovered in 1979 through the detection of repeated bursts from the direction of the Large Magellanic Cloud (LMC; Mazets et al. 1979). The bursts had softer spectra than those of gamma-ray bursts (GRBs), and hence, the source was initially called a “soft gamma repeater (SGR)”. Subsequently, the 7 sec X-ray pulsar 1E 2259+586 was discovered in the galactic supernova remnant CTB 109 (Fahlman & Gregory 1981). This pulsar was unusual in that its X-ray luminosity was larger than the rotation power without any signature of accretion. Therefore, it was initially called an “anomalous X-ray pulsar (AXP)”. Subsequently, more SGRs and AXPs were discovered and were thought to be different types of neutron stars. However, Thompson & Duncan (1995) theoretically unified them using a magnetar model, and Gavriil et al. (2002) indeed discovered SGR like bursts from an AXP (1E 1048.1-5937) subsequently. Hence, the distinction between SGRs and AXPs is blurred observationally as well as theoretically.
A magnetar can be firmly identified only by measuring the spin-inferred magnetic field strength . Historically, the majority of the known magnetars were discovered during their X-ray bursts/outbursts via a targeted search for periodicity when the source was bright. The sensitivity of X-ray observatories has improved significantly, and it may now be possible to discover a relatively faint magnetar serendipitously, even if the magnetar is not in the outburst state. Indeed, several quiescent magnetars have been discovered in this manner (e.g., J164710.2-455216; Muno et al. 2006). Of course, the determination of the magnetic field strength is the most crucial test, but it should be noted that some magnetars are known to have lower magnetic fields (e.g., Rea et al. 2013) than a conventional rotation-powered pulsar (e.g., PSR B1509-58; Wang et al. 2013; Chen et al. 2016). Therefore, we cannot identify a magnetar based on the magnetic field alone. The X-ray spectra of conventional X-ray bright rotationpowered pulsars (e.g., weak or no blackbody emission) differ significantly from those of magnetars, and thus, careful characterization of the spectrum is required.
Finding more magnetars is important for many reasons. The origin and evolution of magnetars, and their relation to other neutron stars are not yet well understood, and thus, population studies with larger samples of magnetars are required. In addition, magnetars are promising targets of future gravitational wave detectors because their strong internal magnetic stress may deform the star (Beloborodov & Li 2016), and such deformed rotating stars emit gravitational waves (Bonazzola & Gourgoulhon 1996).
Although this deformation is estimated to be too small for near-future gravitational detectors to detect their waves, these may be detected as stochastic background or as a point source if the distortion is very large, perhaps during a giant flare period.
In this study, we searched for magnetars among X-ray point sources in the Chandra archival data using a procedure we developed. In Section 2, we report the results of our study in terms of the properties of known magnetars; these properties were used to develop a procedure and automated packages for selecting magnetar candidates. We present the procedure and apply it to archival Chandra data in Section 3 and present our conclusions in Section 4.
2 BASIC PROPERTIES OF KNOWN MAGNETARS
To select potential magnetar candidates for further identification, we need to know the properties of magnetars. This was achieved through a statistical study of known magnetars. The spatial, spectral, and temporal properties of known magnetars are well summarized in the magnetar catalog and in the study by Olausen & Kaspi (2014). We used this catalog and reference to gain a quantitative understanding of magnetar properties and then make our selections. It should be noted that 29 magnetars are listed in the catalog; however, we needed to know their spectral/temporal properties very well to use them to find similar X-ray sources. Therefore, we used the information from only 19 magnetars (from among the 29 listed) of which the spectral and temporal properties were well characterized.
The Chandra database is immense, and we needed to select regions of the sky wherein magnetars were likely to be found. This could be done based on the work of Olausen & Kaspi (2014). They found that magnetars are rather randomly distributed in galactic longitude and lie within 5° from the galactic plane (Figs. 3 and 4; Olausen & Kaspi 2014). Therefore, we used observations made in this region.
The X-ray spectra of magnetars are generally described by a blackbody-plus-power-law (BBPL) model, although the emissions of some magnetars appear to be a simple power law (PL) or a simple blackbody (BB), perhaps because of limited statistics. In some magnetars, strong hard X-ray emissions of >10 keV are observed (Kuiper et al. 2006) and a putative detection of gamma rays was made from a magnetar (e.g., Wu et al. 2013). The latter, if real, will aid in determining the emission geometry (e.g., Harding 2013) and can be compared with various emission scenarios (e.g., An et al. 2015); these properties were not used in our identification procedure because we are using soft X-ray band data (<10 keV). To characterize the general spectral properties, we obtained the spectral parameters from the magnetar catalog and generated distributions of BB temperature (kT) and PL photon index (Γ). Fig. 1 shows the kT and Γ distributions of 12 magnetars with BBPL spectra. For these magnetars, the BB temperature is rather tightly bound (0.36 - 0.58 keV) and the photon index is in the range of 1.6 - 4. We used these ranges for the spectral searches below. The other seven magnetars exhibited a single-component spectrum (BB or PL) for which kT and Γ were in the ranges of 0.12 - 0.5 keV and 1 - 3.45, respectively.
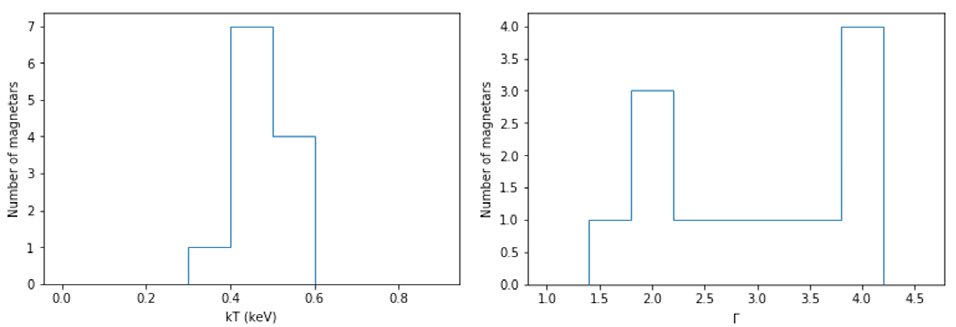
The temporal properties of magnetars are crucial for the final confirmation that an X-ray source is a magnetar, and these should be determined by measuring pulsations (periodic modulation). The general temporal properties of the 19 best-known magnetars are shown in Fig. 2. However, it was unlikely that we would detect pulsations in our study given the limited statistics and temporal baseline. This is because, for the detection of pulsations, the time resolution of the observatory is required to be sufficiently good and the pulsation should be strong. The time resolution for the Chandra X-ray Observatory (CXO) data we used (image mode) is 3.24 sec (full frame, most of the observations) or 0.4 sec (sub-array); thus, at best, sources with a period greater than ~6 sec or 0.8 sec could be detected as pulsating sources. Furthermore, for the typical number of photons collected (~100 photons per source), the pulsed fraction of the potential magnetar should be greater than 30 % for its pulsations to be detected. Among the 19 best-known magnetars, 11 had a period >6 sec and 11 had a pulsed fraction >30 % (see Fig. 2). This implies that the probability that we would detect the pulsations of a source (if it was a magnetar) is only ~33 %. Because the detection of pulsations would almost certainly identify the source (no longer a candidate), we retained such sources in the candidate list even if we did not detect pulsations from them.
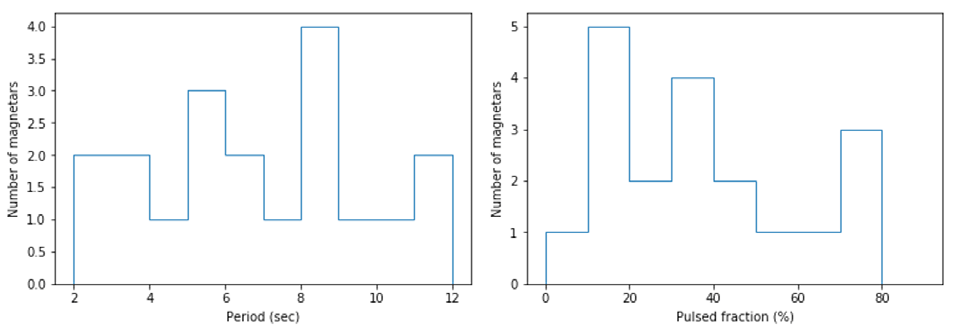
3 OBSERVATIONAL DATA ANALYSIS AND RESULTS
We used the archival data of observations made by CXO between January 2002 and January 2018. The observations were performed with an advanced charge-couple device (CCD) imaging spectrometer (ACIS). The ACIS-I detector consists of six CCDs and provides high-resolution imaging over a ~17' field of view (FOV). The angular resolution is very good with a half-power diameter less than 0.5'', and the time resolution is ~3.2 sec (Garmire et al. 2003). By using a restricted region of the detector, a better time resolution (0.4 sec) can be achieved with the reduction of the FOV. While there are other X-ray observatories (Swift, XMM-Newton, etc.), their angular resolution is not sufficient for this study. Therefore, we only use Chandra data for this study.
We used 1,282 Chandra observations (the typical exposure per observation is 20 ks) downloaded from the High Energy Astrophysics Science Archive Research Center data arch-ive (https://heasarc.gsfc.nasa.gov/cgi-bin/W3Browse/w3browse. pl). These data were reprocessed with the Chandra_repro tool of CIAO (Chandra Interactive Analysis of Observations) 4.9 along with CALDB 4.7.6, and we analyzed the data following a procedure we developed, which is described below.
Based on the general properties of the best-known magnetars (Section 2), we developed the following procedure for magnetar searches:
① We found point sources in the Chandra image. We selected point sources because magnetars are isolated neutron stars. Although we could have used the existing Chandra catalog (Evans et al. 2010) directly, we chose to reanalyze the data for a more accurate determination of the spectral parameters required in other steps (customized spectral models in step ②) and the position of each X-ray source in step ③. Because the number of detected events from a source is required to be sufficiently large for spectral characterization, we ignored sources with <30 detected events.
② For the point sources found in step ①, we fitted the spectra using a BB, PL, and BBPL model to measure the spectral parameters. We then selected sources with a BB temperature (kT) in the range 0.36 - 0.58 keV and PL photon index (Γ) in the range of 1.6 - 4 for the BBPL. These are typical magnetar emissions (Section 2). In addition, we separately collected sources with kT in the range of 0.12 - 0.5 keV for BB emission or Γ in the range of 1.0 - 3.45 for PL emission.
③ We searched optical and infrared (IR) catalogs for bright optical sources near the X-ray sources. It was required that there be no bright optical source within a specified error circle for each source. The size of the error circle was determined by the uncertainty of the source position (step ①) and of the CXO absolute astrometry (~1” at 99 % confidence). These two uncertainties were added in quadrature. This was to ensure that the X-ray source had no bright optical counterpart with 3σ confidence because magnetars are very faint in the optical/IR band (>20 mag). For this, we used the NOMAD and 2MASS catalogs (Zacharias et al. 2004; Skrutskie et al. 2006). It should be noted that the position uncertainties in these catalogs are negligible as compared to the CXO uncertainties.
④ We performed a timing analysis to detect pulsation. Detection of pulsation would be strong evidence that the X-ray source is a magnetar, although a final confirmation would need to be obtained by measuring the period derivative (i.e., magnetic field strength). In addition, long term variability (e.g., outburst tail) may aid in identifying a candidate, but in general, the fields are not observed multiple times. Therefore, we do not consider this there.
For reference, we applied this procedure to the known magnetars in the catalog to estimate the probability of discovering a magnetar if there was a real magnetar in the field. Four of the 19 magnetars passed all four steps, and thus, the obtained probability was 21 %. If we assume that the spectral/temporal properties of magnetars are all independent, the probability would be 16 % (14/19 for step ①, 12/19 for step ②, 19/19 for step ③, and 11/19 × 11/19 for step ④).
Data analysis was performed for each of the steps in the following manner. For step ①, we used the “wavedetect” tool of CIAO, which finds X-ray sources based on a wavelet decomposition technique and reports the best position and uncertainty. For each of these sources, we extracted events in the 0.5 – 10 keV band within a circular region with a radius of 3'' centered at the source position for the source spectrum, and an annular region with an inner radius of 3'' and an outer radius of 5'' for the background spectrum. Spectral response files were generated separately for each source using the “specextract” tool of CIAO, and each spectrum was fitted using the BBPL, PL, and BB models in XSPEC3. We used the tbabs photoelectric absorption model in XSPEC with the abundance table of Anders & Grevesse (1989) and photoelectric cross-sections from Verner et al. (1996). We note that it is optimal to use an elliptical region to maximize the signal-to-noise ratio for off-axis sources, but 3'' is sufficiently large for the majority of the sources we find (at off-axis angles <6').
For the sources that passed the spectral test, we checked for optical or IR counterparts using the NOMAD and 2MASS catalogs. We searched for nearby optical sources in the catalogs and calculated the angular separation between the X-ray and optical sources, where and are the positions of the X-ray and optical/IR sources. The uncertainty of the separation was calculated by summing the individual uncertainties in quadrature where and are the position uncertainties for the X-ray and optical/IR sources). Because Chandra images have an absolute astrometry uncertainty of 1''(3σ), we added this to the position uncertainties in quadrature () where Chandra absolute astrometric accuracy). The significance for coincidence Ks was then calculated using , and we selected X-ray sources with Ks > 3 for any nearby optical/IR sources.
Finally, we performed a timing analysis for the sources that passed steps ①–③ using the Z21 test (Buccheri et al. 1983) to find any periodic modulation. Because of the limited time resolution, we tested for periodicity in the range of 5 - 15 sec (for observations with a 3.24 sec time resolution) or 0.8 - 15 sec (for observations with a 0.4 sec time resolution). We scanned this range with the time-step ΔT=10−7 and then calculated the Z21 value for each test period. The Z21 test is particularly useful because the distribution function is known; Z21 values are known to follow the χ2 statistic with two degrees of freedom. The larger Z21 is, the more likely that the source is pulsating. We calculated the significance for the maximum Z21 in the scan range for each source while correcting for the trial factor.
Because the data set we were analyzing was very large and included several X-ray sources, we created a python package to automate the analysis procedure. Each step of the procedure (in Section 3.1) was implemented in the package, and after each step, the obtained intermediate results were recorded in a file.
After setting up the search procedure, we verified it using a known magnetar. We analyzed a 40 ks Chandra observation (Obs. ID 5411) that has the magnetar CXOU J164710.2- 455216 (J1647 hereafter) in its field. We first searched for X-ray sources in the observation using the “wavedetect” tool and found J1647 (Fig. 3 top left). The best source position was R.A. = 16h 47m 10.199s, Decl. = −45° 52' 17.33'' with a position uncertainty of 1'', which is consistent with the catalog values (R.A. = 16h 47m, 10.2s, Decl. = -4°52′ 17.33″). The source spectra were fitted with a BB (kT=0.49keV), a PL (Γ = 3.28), and a BBPL model (kT=0.53 keV, Γ=3.3). The best-fit spectral parameters were well within the range we set for magnetar candidates in Section 3.1 (step ②). Next, we confirmed that this magnetar had no bright optical or IR counterpart (Fig.3 bottom left) and that the source was pulsating (Fig.3 bottom right). As expected, we identified this magnetar as a good magnetar candidate using the procedure and the analysis package we developed, thereby verfying them.
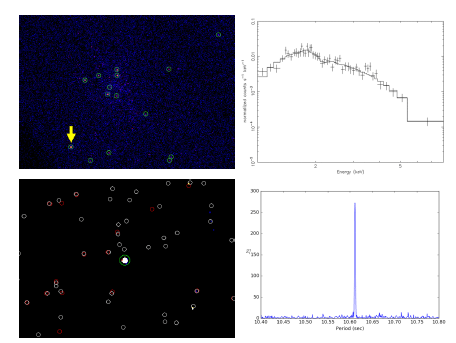
The field we studied was very large and had within it six known magnetars (IE 1547.0-5408, CXOU J171405.7-381031, SGR J1745-2900, SGR 1806-20, SGR 1833-0832, and SGR 1900+14). All these were found in the new imaging analysis. The X-ray spectra of these magnetars fit well with the BBPL model. None of them had an optical or IR counterpart. Hence, these magnetars would have become candidates even if they were not already known to be magnetars (Table 1).
![]() |
In the subsequent timing analysis, the pulsation of SGR 1833-0832 was detected with a spin period of 7.5654 sec, which was consistent with that measured by Esposito et al. (2011). Thus, we could identify this one as an “almostcertain” magnetar, thereby again verifying the procedure and packages, as in the case of J1647. However, the pulsation of the other magnetars was not detected. This was owing to the short period or perhaps to the small pulsed fraction (Mereghetti et al. 2006; Sato et al. 2010; Dib et al. 2012; Kaspi et al. 2014). For example, SGR 1806-20 is very bright but has a very small pulsed fraction (~2.5 %; Woods et al. 2007). The pulsed fraction is further reduced when blurred owing to the Chandra time resolution of 3.24 sec. Hence, the pulsation of SGR 1806-20 was not detected despite having many collected events. In Table 1, we summarize the results of each step for six magnetars.
After all the known magnetars in the field were studied, we inspected other sources. In our image analysis, we found 32,838 point sources with known positions. We fitted the spectrum of each of the sources with a BBPL, a BB, and a PL model and found that 149 (BBPL), 3,109 (BB), and 10,744 (PL) sources, respectively, passed step ②. In Fig. 4 (left), we present the best-fit kT and Γ for 135 sources with spectra that are fitted to the BBPL model. The results for single-component models (a PL or a BB) are shown in Fig. 4 (middle and right). It should be noted that some sources are in multiple spectral categories. Next, we checked if the X-ray sources have an optical/IR counterpart and eliminated those with a counterpart. This left 25 (BBPL), 811 (BB), and 1,725 (PL) X-ray sources. The analysis results are summarized in Table 2.
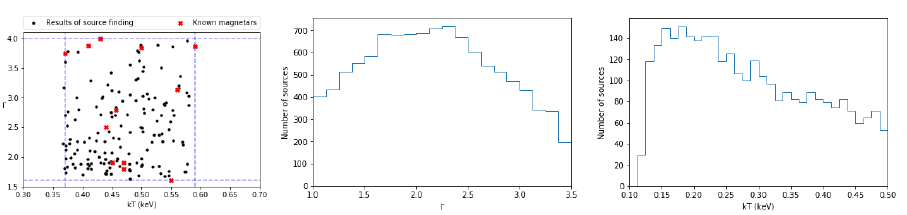
![]() |
Finally, we performed a timing analysis. The maximum Z21 value for the sources that passed steps ①–③ was 31.54, which corresponded to the null hypothesis probability of 1.41×10−7. This is small; however, considering the trial factor of 108, this is not significant. In conclusion, we did not find significant pulsations in any of the sources.
Theoretically, the spectrum of a magnetar should be modeled using a BBPL model (Beloborodov 2013), and indeed, the majority of magnetars have a two-component emission. Therefore, we might find better candidates by investigating the 25 sources with a two-component emission. We list these separately in Table 3. In this table, we also report the best-fit parameters with the uncertainty. The parameters were not very well constrained for these faint sources. For very faint sources, some parameters were not constrained. In this case, we maintained those parameters fixed to derive uncertainties for the other parameters. Because some of the sources might already have been studied, we searched the SIMBAD catalog (https://simbad.u-strasbg.fr/simbad) to check if any of the sources had been previously identified. We found that XMMU J173203.3-344518 (hereafter, J1732) in the table was previously identified as a potential pulsar in a supernova remnant (Halpern & Gotthelf 2010a). There are some other sources listed in the CXO or XMM catalog, but their nature is not yet clear.
4 DISCUSSION AND CONCLUSIONS
We developed a new procedure to search for magnetar candidates. The procedure was implemented in a python package that could analyze a large data set automatically. The package can be used to reduce the number of targets (good magnetar candidates) in more detailed searches of current and future X-ray satellite data. We used the package to analyze 1,282 Chandra observations and selected 25, 811, and 1,725 magnetar candidates, using a BBPL, BB, and PL spectrum, respectively. Unfortunately, pulsation was not found in the case of any of the sources.
We verified the procedure and software package using a known magnetar: J1647 was confirmed using the new procedure. In addition, we identified one among six previously known magnetars in our survey field. This implies a detection probability of 10 - 47 % (68 % confidence range between 16 % and 84 % percentiles) assuming a Poisson distribution. This detection probability is consistent with those (21 % or 16 %; see Section 3.1) we estimated using the 19 best-known magnetars. This reconfirmed that the procedure and software package work properly.
We did not identify pulsation in the case of any of the sources. Should there have been a real magnetar in the list (Table 3), there is a 16 % probability that we would have discovered it. With this probability, we can estimate the number of potential magnetars in the survey; as no magnetar was discovered using our method, we infer that there are less than 14 magnetars amongst the considered candidates with 90 % confidence. That is, if there were more than 14 magnetars in our survey, there is a 90 % chance we would have discovered one (or more).
With respect to the 25 sources with BBPL emission (Table 3), it is likely that not all of them would be magnetars. Some of them would be isolated pulsars, low-mass pulsar binaries, or pulsars in pulsar wind nebulae. These types of pulsars can have BBPL emissions with no bright optical counterpart. Because X-ray pulsars are important astrophysical objects, further optical and X-ray observations and analysis of the archival data of these sources will be interesting even if they are not magnetars.
![]() |
It is difficult to determine what the sources with a singlecomponent emission are. These sources are very faint, and their X-ray spectra are not constrained. A spectrum of any faint X-ray source would be fitted to a BB or PL model. Therefore, we did not list them separately. As optical and X-ray survey goes deeper, we should be able to identify them better using our automated package.
Our survey is incomplete because we used only a subset of the archival Chandra data and optical catalogs and because the time resolution of ACIS-I is 3.2 sec, which is insufficient for the detection of a magnetar with a period of less than ~6 sec. Only a small number of observations are obtained with a 0.4 sec time resolution. Moreover, very faint sources were excluded from the search. Further studies of the currently available and future X-ray data will aid in identifying more magnetars and other interesting X-ray sources.